Test 20 items
- Some normal values
- What happens during exercise
- Ventilation perfusion
- Vq ratio; what is zero and infinity
- Bohr equation and application of copd
- Process of co2 transport to tissue
- Factors that affect gas diffusion
- Recalls on partial pressures
- What happens during exercise
- Hgb dissociation curve (2 questions)
- Factor of shift to left or right
- function of 2,3-dpg/bpg
- Haldane and Bohr effect
Contents
- 0.0.1 1. ガス交換の場所
- 0.0.2 2. 呼吸膜(Respiratory Membrane)または肺膜(Pulmonary Membrane)
- 0.0.3 3. 拡散(Diffusion)によるガス交換
- 0.0.4 図1の解説
- 0.0.5 臨床的重要性(Clinical Importance)
- 0.0.6 毛細血管の通過時間(Capillary Transit Time)
- 0.0.7 拡散制限型と灌流制限型のガス交換
- 0.0.8 呼吸膜の拡散能(Diffusing Capacity of Respiratory Membrane)
- 0.0.9 測定方法
- 0.0.10 シャント(Shunt)
- 0.0.11 V/Q不均等(V/Q Defects)
- 0.0.12 V/Q比の変化(Changes in V/Q Ratio)
- 0.0.13 ガス輸送(Gas Transport)
- 0.0.14 1. 大気圧とダルトンの法則
- 0.0.15 2. 吸気中の酸素分圧(PIO2)
- 0.0.16 3. 肺胞-動脈酸素分圧勾配(A-a Gradient)
- 0.0.17 4. 肺胞ガス方程式(Alveolar Gas Equation)
- 0.0.18 5. A-a勾配の増加原因
- 0.0.19 1. 溶解型酸素(Dissolved O2)
- 0.0.20 2. ヘモグロビン結合型酸素(O2 Bound to Hemoglobin)
- 0.0.21 3. ヘモグロビンの異常型および変異型
- 0.0.22 酸素の輸送:肺から体組織へ
- 0.0.23 ヘモグロビン-酸素解離曲線(Oxygen-Hemoglobin Dissociation Curve)
- 0.0.24 呼吸器内でと末梢組織での酸素の動態
- 0.0.25 酸素の「バッファー」作用
- 0.0.26 大気中の酸素濃度の変化
- 0.0.27 ヘモグロビン–酸素解離曲線の変化
- 0.0.28 細胞内の酸素代謝への影響
- 0.0.29 二酸化炭素(CO2)の血液中での輸送
- 0.0.30 低酸素症(Hypoxia)
- 1 CHAPTER 40
1. ガス交換の場所
肺には約300百万個の肺胞(alveoli)があり、これがガス交換の主な場所です。各肺胞の直径は約0.2 mmで、肺胞の壁は非常に薄く作られています。このため、肺胞内の空気と肺毛細血管(pulmonary capillaries)は非常に近接しており、効率的にガス交換が行われます。
ガス交換は呼吸単位(respiratory unit)を通じて行われ、これは以下の部分で構成されています:
- 呼吸細気管支(respiratory bronchiole)
- 肺胞管(alveolar ducts)
- 肺胞(alveoli)
2. 呼吸膜(Respiratory Membrane)または肺膜(Pulmonary Membrane)
呼吸膜はガス交換が行われる場所で、以下の層で構成されています:
- 肺胞を覆う薄い液体の層(a thin layer of fluid)
- 肺胞上皮細胞(alveolar epithelial cells)
- 上皮細胞の基底膜(basement membrane of epithelial cells)
- 肺胞上皮と毛細血管膜の間の薄い間質(a thin interstitial space)
- 毛細血管の基底膜(capillary basement membrane)
- 毛細血管の内皮(capillary endothelium)
呼吸膜の平均的な厚さは約0.5 μm(マイクロメートル)で、範囲は0.2 μmから0.6 μmです。呼吸膜の総表面積は、健康な成人男性で約70 m²です。ただし、**間質性浮腫(interstitial edema)や肺の線維化(fibrosis of the lungs)**では膜の厚さが増加することがあります。また、**肺切除術(pneumonectomy)や肺気腫(emphysema)**のように、肺胞の破裂により呼吸膜の表面積が減少することがあります。
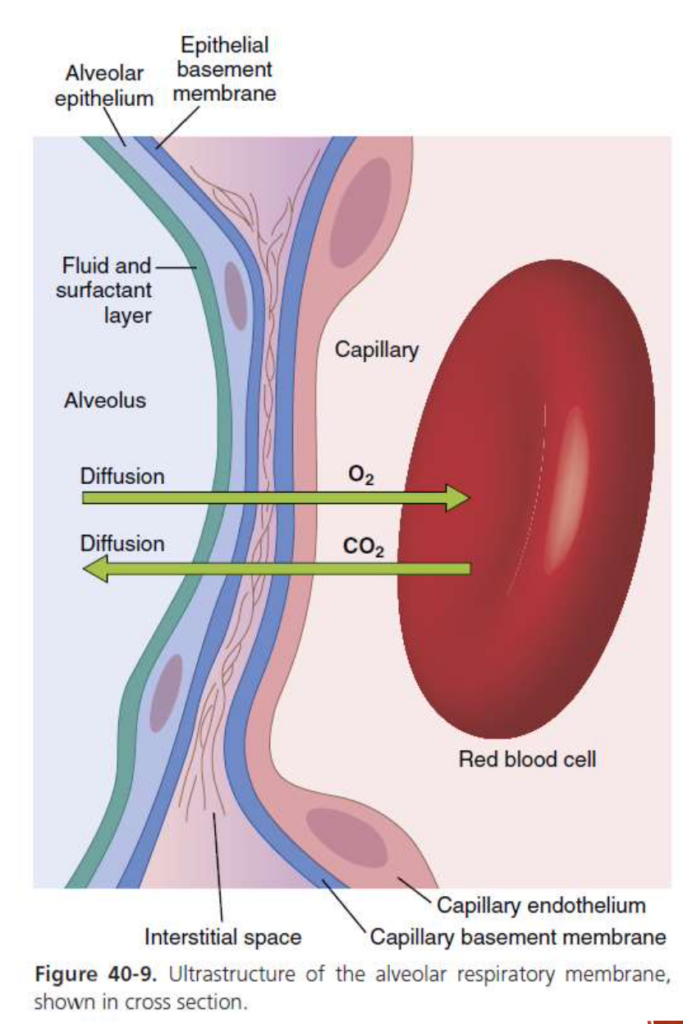
3. 拡散(Diffusion)によるガス交換
ガスは呼吸膜を通じて拡散しますが、この過程にはいくつかの重要な要因が影響を与えます。拡散に影響を与える要因は以下の式で説明されます:
拡散量 = (ΔP × A × S) / (d × √M)
ここで、
- ΔP: 拡散経路の両側の分圧差(partial pressure difference)
- A: 拡散経路の断面積(cross-sectional area)
- S: ガスの溶解度(solubility of the gas)
- d: 拡散距離(distance of diffusion)
- M: ガスの分子量(molecular weight of the gas)
それぞれのガスの特性は溶解度(S)と分子量(M)によって決まり、この2つの要因がガスの拡散係数(diffusion coefficient)を決定します。拡散係数はS/√Mに比例します。
図1の解説
図1では、ガスが肺胞-毛細血管膜を横断して移動する様子が示されています。この移動は、以下の要素に依存します:
- 膜面積(membrane area)
- 膜の厚さ(membrane thickness)
- ガス分子の分子量(molecular weight)
- ガス分子の溶解度(solubility)
- 肺胞-毛細血管膜の両側の圧力勾配(pressure gradient)
以上がガス交換の基本原理となります。
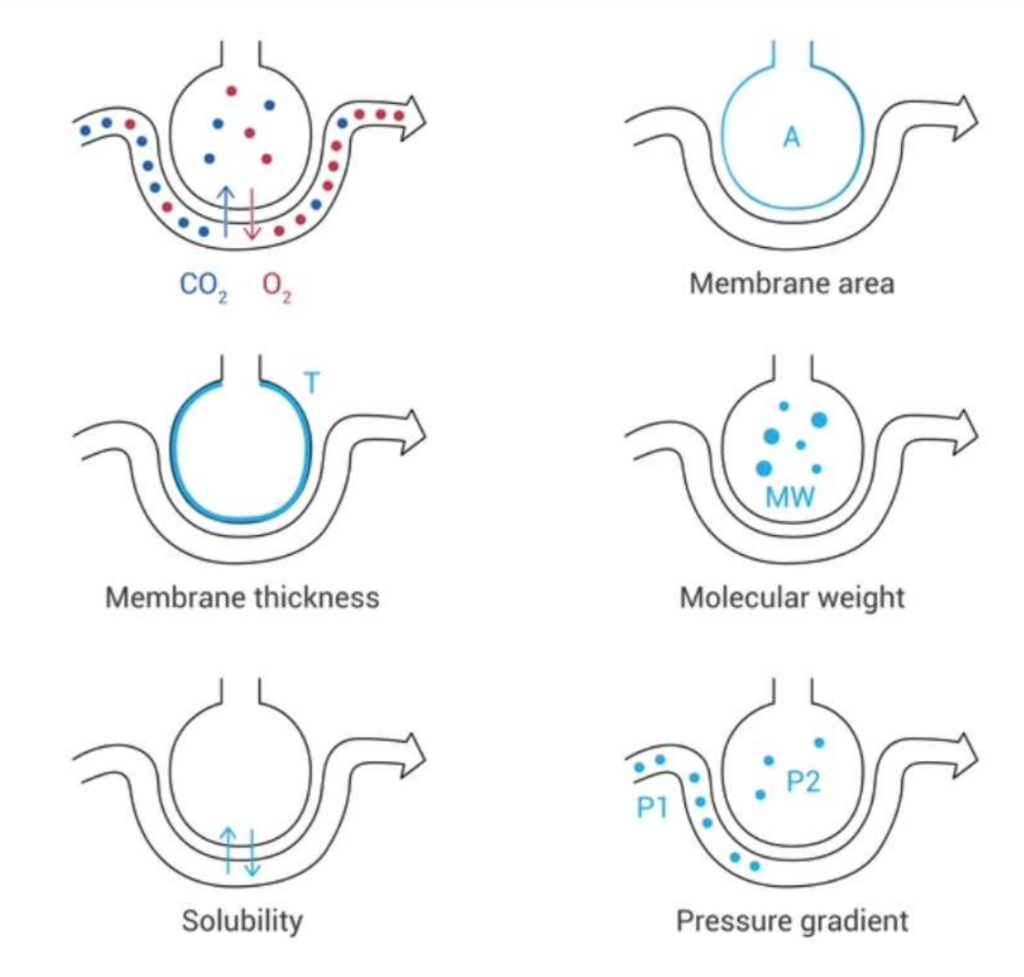
臨床的重要性(Clinical Importance)
- CO2の拡散係数(Diffusion Coefficient for CO2)はO2の約20倍
これは、同じ分圧レベル(partial pressure levels)でCO2がO2よりも約20倍速く呼吸膜(respiratory membrane)を拡散することを示しています。このため、肺疾患では低酸素血症(hypoxemia)(血液中の酸素不足)が発生しやすく、高炭酸血症(hypercarbia)(CO2の蓄積)はまれです。CO2は非常に効率的に拡散するため、CO2が体内に蓄積することはあまりありません。 - 肺気腫(Emphysema)などの疾患ではガス拡散が減少
肺胞の破壊により、呼吸膜の有効な表面積(A)が減少するため、ガス交換能力が低下します。 - 拡散距離(d)の増加による拡散の減少
急性肺損傷(acute lung injury)の浮腫や、間質性肺疾患(interstitial lung diseases)の線維化(fibrosis)では、拡散距離が増加し、ガス交換がさらに阻害されます。
毛細血管の通過時間(Capillary Transit Time)
- 赤血球が肺毛細血管を通過する時間は、肺毛細血管の末端PO2(酸素分圧)および拡散能を決定する重要な要素です。
- 安静時の心拍出量(cardiac output)では、赤血球が肺循環を通過するのに約4~5秒かかり、このうち約0.75秒(約0.8秒)が肺胞毛細血管(alveolar capillary)内で過ごされます。
- 肺循環内の血液量は約450 mLで、このうち約70 mL(範囲60〜140 mL)が肺毛細血管に存在します。

拡散制限型と灌流制限型のガス交換
- 灌流制限型ガス交換(Perfusion-limited Gas Exchange)
- ガス交換は**血流速度(rate of blood flow)**に制限されます。O2、CO2、N2Oなどのガスは、血液-空気障壁(blood-air barrier)を自由に拡散できます。
- 血漿中のガス濃度は、血液が毛細血管の端に達する前に肺胞内の濃度と等しくなります。
- 血流が増加すればガス交換も増加します。
- 正常条件(安静時)では、このタイプのガス交換が見られます。
- 例: 運動中の筋肉でのガス交換。運動中は血液の流れが速くなり、筋肉での酸素需要が増加します。筋肉の毛細血管での酸素交換が十分に行われる前に血液が流れてしまい、ガス交換が灌流によって制限される場合があります。
- 拡散制限型ガス交換(Diffusion-limited Gas Exchange)
- ガス交換は、血液-空気障壁を越えるガスの**拡散速度(diffusion rate)**に制限されます。例えばO2やCOなどのガスがこの例です。
- 血漿中のガス濃度は、毛細血管の終端に達しても平衡に達しません。
- この状況は、激しい運動時や肺気腫、**肺線維症(lung fibrosis)**のような病的状態で発生します。
- 例: 肺線維症(肺の線維化)などの肺の病気。線維化により肺の組織が厚くなり、ガスが肺胞から血液へ拡散するのが困難になります。結果として、酸素が血液中に十分に取り込まれず、拡散制限型のガス交換が生じます。
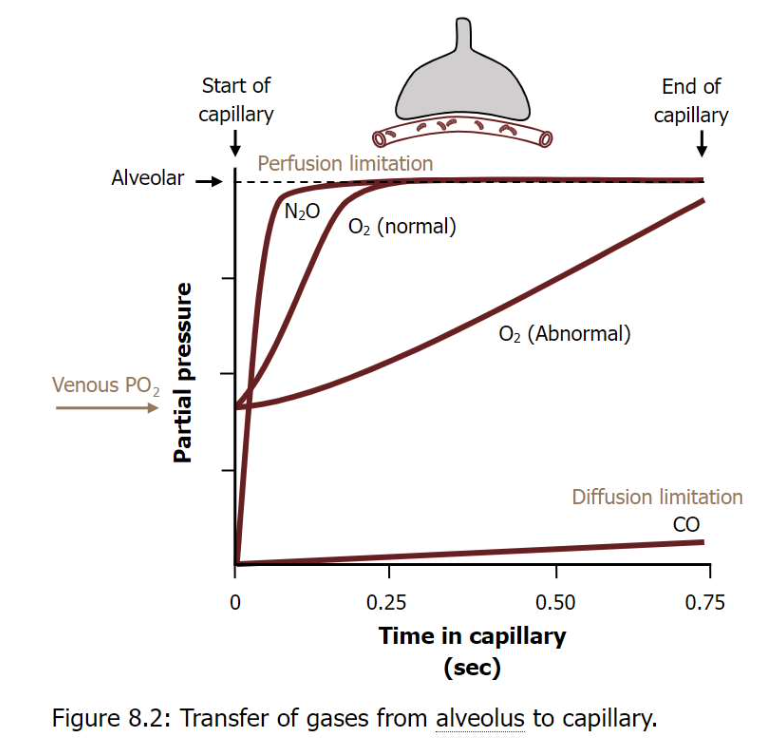
呼吸膜の拡散能(Diffusing Capacity of Respiratory Membrane)
- 呼吸膜の拡散能とは、分圧差が1 mmHgの条件で、1分間にどれだけのガスが膜を通じて拡散するかを定義します。
- 呼吸膜の拡散能は、呼吸膜の**表面積(surface area)**に比例し、**厚さ(thickness)**に反比例します。
- CO(炭素一酸化物)の拡散能(DLCO)は、拡散制限型のガス交換の指標として測定されます。
- 正常時のDLCOは安静時で17 mL/分/mmHgです。
- O2の拡散能はCOの1.23倍で、これはO2の拡散係数がCOの1.23倍だからです。O2の拡散能は21〜25 mL/mmHg/分です。
測定方法
- **シングルブレス法(Single-breath method)**が最も一般的なDLCO測定法です。
患者は、0.3%のCOと10%のヘリウムを含むガス混合物を吸入します。この方法で、血液中のCOの取り込みと平均肺胞CO分圧を計算できます。
これにより、O2およびCO2の拡散のメカニズムが、肺の健康状態や運動、疾患によってどのように影響を受けるかを理解することができます。
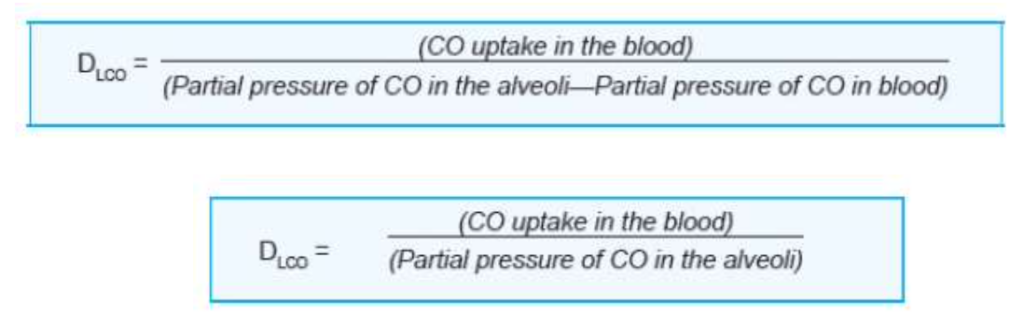
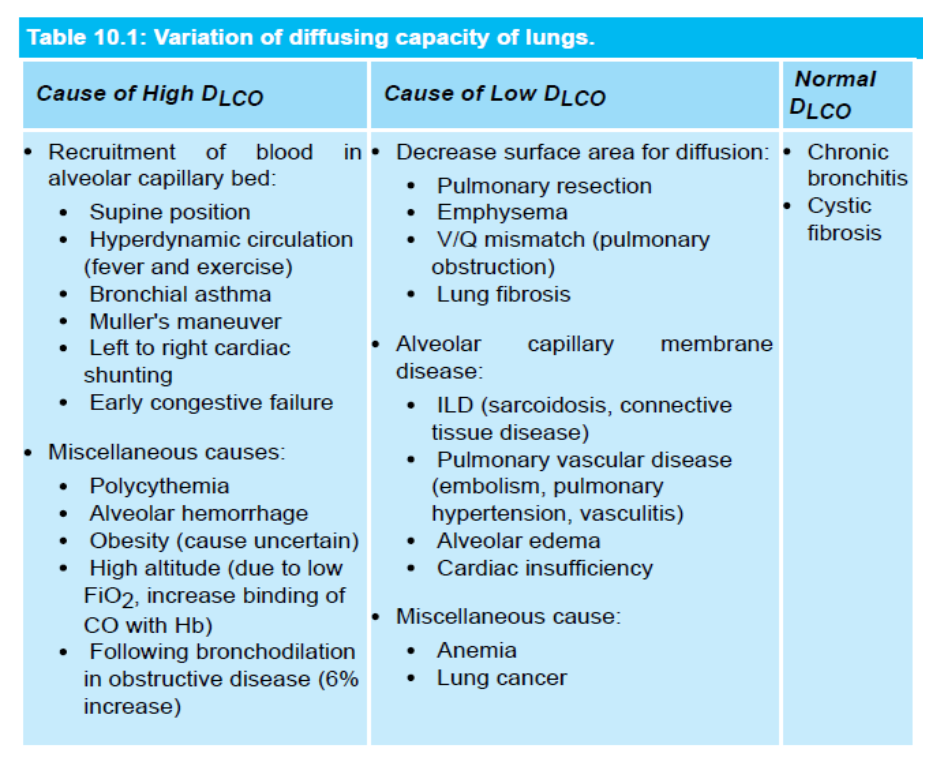
シャント(Shunt)
シャントとは、心拍出量や血流の一部が正常な経路から逸れて他のルートに流れる現象を指します。
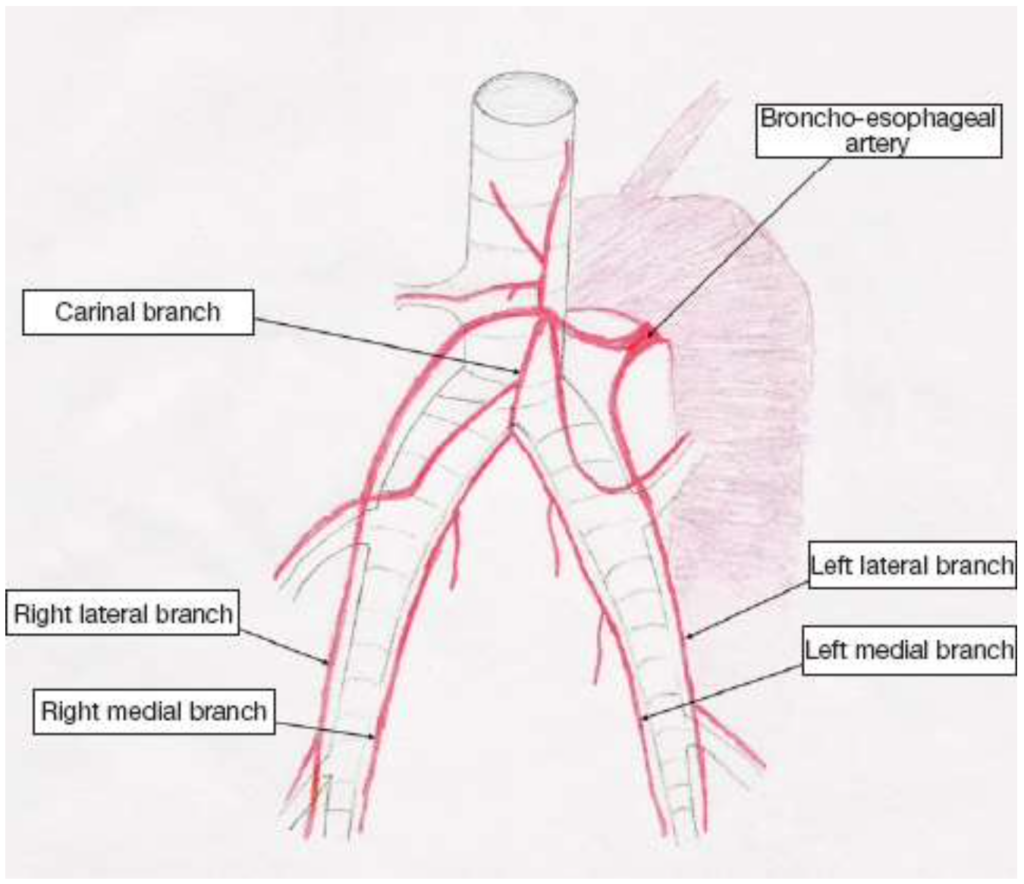
1. 生理学的シャント(Physiologic Shunt)
- 気管支血流(bronchial blood flow): 約2%の心拍出量が肺胞(alveoli)をバイパスして気管支の代謝機能を維持します。これは右から左への生理学的シャントです。
- テーベシウス静脈(Thebesian veins): 冠血流(coronary blood flow)の一部が直接左心室に流れ込み、肺を通らない血液が存在します。
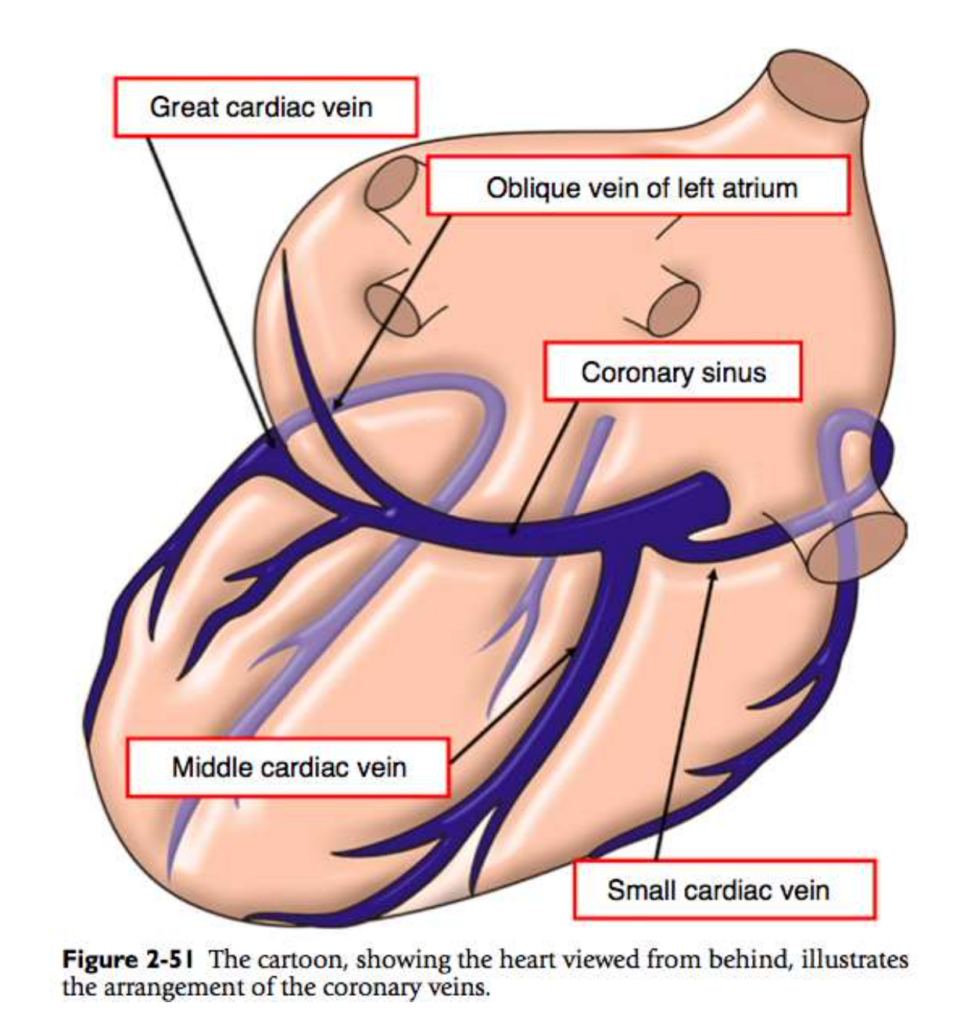
2. 右から左へのシャント(Right-to-left Shunts)
- 右心室から左心室へのシャントが発生すると、心室間の壁に欠損がある場合に見られます。
- **低酸素血症(hypoxemia)**が必ず起こり、100%酸素を吸入しても改善されません。肺を通らないため、酸素化が行われない血液が体循環に流れ込みます。
3. 左から右へのシャント(Left-to-right Shunts)
- 左から右へのシャントはより一般的ですが、通常は低酸素血症を引き起こしません。
- 例として、動脈管開存症(patent ductus arteriosus)や外傷があります。この場合、肺から戻った酸素化された血液が直接右心に加わり、体組織に供給されることなく右心系に戻ります。そのため、右心側の酸素分圧(PO2)は上昇します。
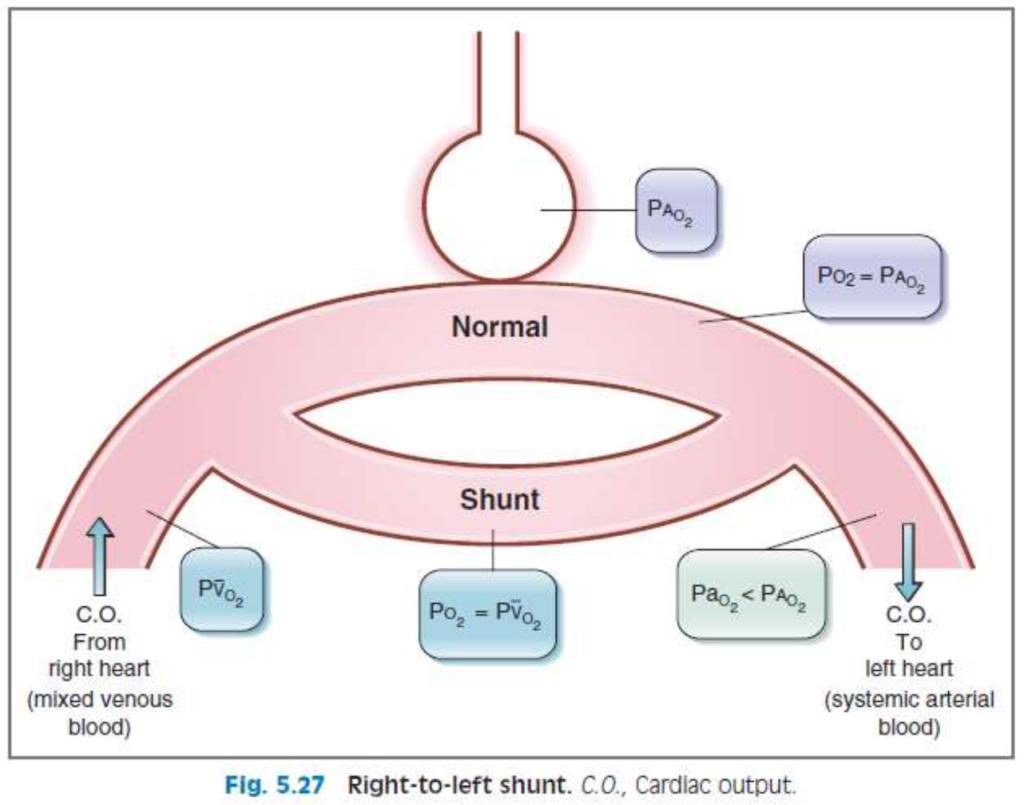
V/Q不均等(V/Q Defects)
1. V/Q比(V/Q Ratio)
- V/Q比は、**肺胞換気量(V)と肺血流量(Q)**の比率です。理想的なガス交換にはこの比率が重要です。
- 呼吸率、1回換気量、心拍出量が正常であれば、V/Q比は約0.8となり、これにより動脈血中の酸素分圧(PO2)は100 mmHg、二酸化炭素分圧(PCO2)は40 mmHgに達します。
2. 肺内の異なる部位でのV/Q比の違い
- **換気(ventilation)と血流(perfusion)**は、立位の正常な肺では均等に分布していません。
- **血流(灌流)は肺の頂部(apex)**で最も低く、**基底部(base)**で最も高くなります。これは重力による動脈圧の影響です。
- 換気も同様に、頂部で低く、基底部で高くなりますが、血流ほどの差はありません。
3. 肺の異なる部位でのV/Q比の違いと酸素・二酸化炭素の分圧
- V/Q比は肺の頂部で高く、基底部で低いです。これにより、酸素と二酸化炭素の分圧にも差が生じます。
- 肺の頂部(高V/Q比)では、酸素分圧(PO2)が高く、二酸化炭素分圧(PCO2)が低いです。これはガス交換が効率的であるためです。
- 肺の基底部(低V/Q比)では、酸素分圧(PO2)が低く、二酸化炭素分圧(PCO2)が高いです。これはガス交換が非効率的であるためです。
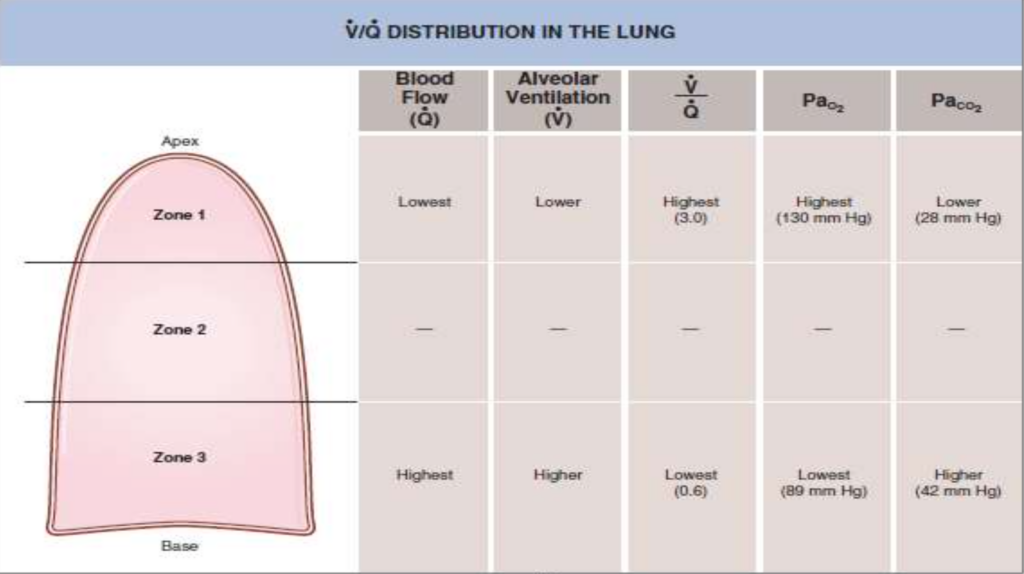
V/Q比の変化(Changes in V/Q Ratio)
1. 気道閉塞時のV/Q比(V/Q Ratio in Airway Obstruction)
- 気道が完全に閉塞された場合(例:ステーキの一部が気管に詰まるなど)、**換気(ventilation)**はゼロになります。
- 血流が正常な場合、V/Q比は0になり、これを**シャント(shunt)**と呼びます。
- 換気されないが血流がある肺ではガス交換が行われないため、肺胞毛細血管血液(pulmonary capillary blood)の酸素分圧(PO2)と二酸化炭素分圧(PCO2)が静脈血の値に近づきます。
- 結果として、**A–a勾配(Alveolar-arterial gradient)**が増加します。
2. 肺塞栓症時のV/Q比(V/Q Ratio in Pulmonary Embolism)
- 肺への血流が完全に遮断された場合(例:肺動脈が塞栓によって閉塞される)、**血流(perfusion)**はゼロになります。
- 換気が正常であれば、**V/Q比は無限大(∞)となり、これを死腔(dead space)**と呼びます。
- 換気されるが血流がない肺ではガス交換が行われないため、肺胞ガスのPO2とPCO2は吸気中の値に近づきます。
まとめ
- シャント(Shunt): 換気がゼロで血流がある場合、V/Q = 0
- 肺胞死腔(Alveolar Dead Space): 血流がゼロで換気がある場合、V/Q = ∞
ガス輸送(Gas Transport)
1. 記号の使用
- P: 分圧(Partial Pressure)
- I: 吸気(Inspired Air)
- E: 呼気(Expired Air)
- A: 肺胞(Alveolar)
- a: 動脈血(Arterial)
- v: 静脈血(Venous)
- 例:PIO2は吸気中の酸素分圧
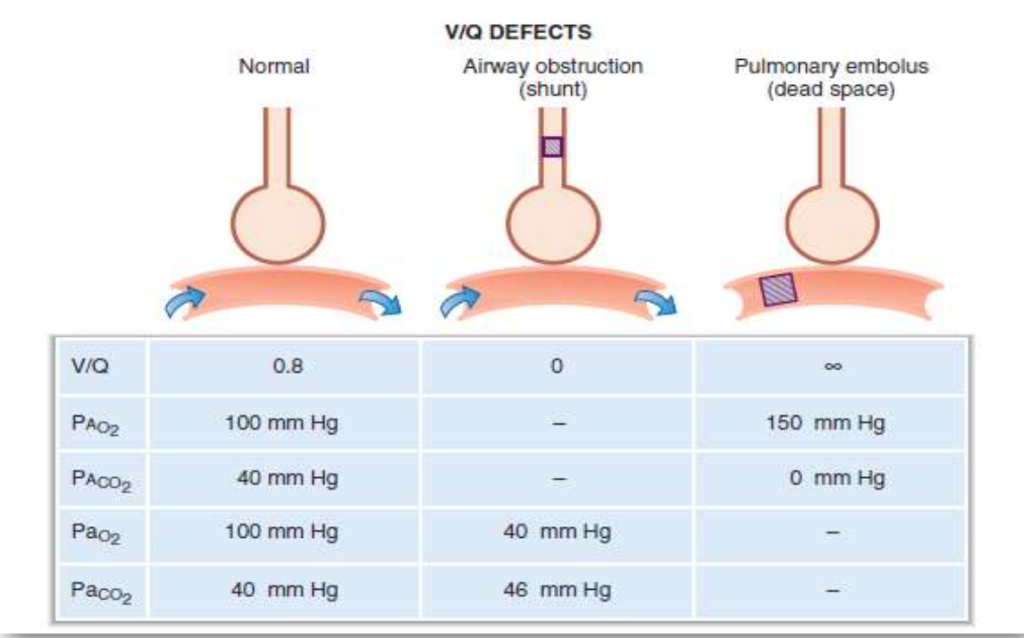
1. 大気圧とダルトンの法則
- 大気圧(Pbaro)は、海面レベルでは760 mmHgです。ダルトンの法則によると、この760 mmHgは空気中のさまざまなガス(酸素、窒素、二酸化炭素など)の分圧の合計で成り立っています。
- 空気中の約**21%**が酸素であるため、海面レベルでの酸素の分圧(PO2)は次のように計算できます:
- 760 × 0.21 = 160 mmHg
2. 吸気中の酸素分圧(PIO2)
- 肺に吸い込まれた空気は気道で温められ、加湿されます。この際、水蒸気分圧(PH2O)が37°Cで47 mmHgとなります。これを考慮して、吸気中の酸素分圧(PIO2)は次のように計算されます:
- PIO2 = (760 – 47) × 0.21 = 149 mmHg
- PIO2 = (760 – 47) × 0.50 = 357 mmHg
このように、酸素濃度が上がることで酸素分圧も大幅に増加します。
3. 肺胞-動脈酸素分圧勾配(A-a Gradient)
- A-a勾配は、肺胞内の酸素分圧(PAO2)と動脈血中の酸素分圧(PaO2)の差です。A-a勾配は、肺でのガス交換の効率を測る指標で、正常では約5–10 mmHgです。しかし、何らかの障害があるとこの勾配が大きくなります。実例:
- **肺塞栓症(Pulmonary Embolism)**の場合、血流が遮断されるため、換気されているのにガス交換が起こらない領域(デッドスペース)ができます。これにより、PAO2は正常でも、PaO2が低下し、A-a勾配が増加します。
- 肺炎(Pneumonia)では、肺胞が炎症により液体で満たされるため、換気が妨げられ、ガス交換が低下します。これにより、PAO2は正常でもPaO2が低下し、やはりA-a勾配が増加します。
4. 肺胞ガス方程式(Alveolar Gas Equation)
- PAO2は、次の式で計算されます:
- PAO2 = PiO2 – (PaCO2 / R)
- PiO2 = 吸気中の酸素分圧(約150 mmHg)
- PaCO2 = 動脈血中の二酸化炭素分圧
- R = 呼吸商(通常0.8)
- PaCO2が50 mmHgの場合、PAO2はPAO2 = 149 – (50 / 0.8) = 87 mmHg
これにより、酸素供給が減少し、A-a勾配が増加します。
5. A-a勾配の増加原因
- 年齢: 加齢に伴い、肺の弾力が減少し、A-a勾配が自然に増加します。
- 高濃度酸素吸入: 酸素療法で100%酸素を吸入すると、一時的にA-a勾配が増加します。
- 右から左へのシャント: 心臓や肺の異常により、酸素化されていない血液が直接左心房に流れると、PaO2が低下し、A-a勾配が増加します。
- V/Qミスマッチ: 肺塞栓や無気肺などでは、換気と血流のバランスが崩れ、ガス交換が効率的に行われなくなります。


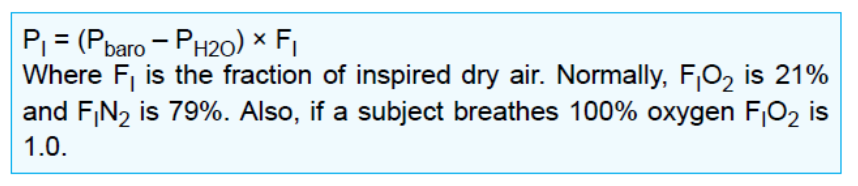
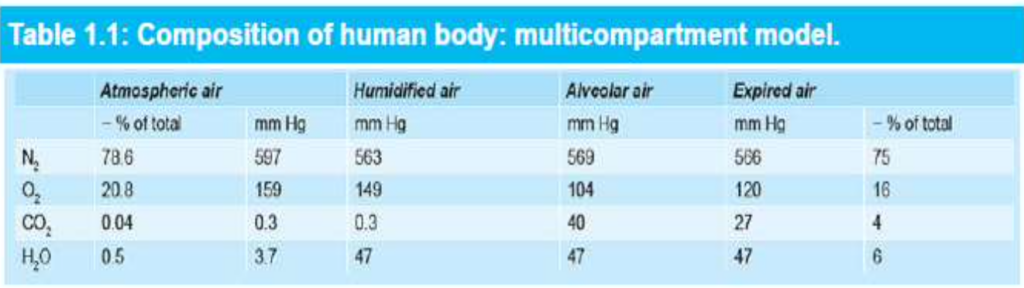
1. 溶解型酸素(Dissolved O2)
- **血液中の酸素の約2%**は自由に溶解した形で存在します。
- この形態の酸素は**酸素分圧(PO2)**を生じさせます。つまり、血中で分子として溶けている酸素が圧力を生み出すため、動脈血ガス分析で測定される酸素分圧はこの溶解型酸素に由来します。
- ヘンリーの法則(Henry’s law)に従って、溶解型酸素の濃度は酸素分圧に比例します。比例定数は酸素の溶解度で、血液中での酸素の溶解度は0.003 mL O2/100 mL 血液/mmHgです。
- 例えば、動脈酸素分圧(PaO2)が100 mmHgの場合、溶解型酸素の濃度は0.3 mL O2/100 mLとなります。
2. ヘモグロビン結合型酸素(O2 Bound to Hemoglobin)
- 血液中の酸素の**約98%は、赤血球内のヘモグロビン(Hemoglobin, Hgb)**に結合した形で存在します。
- ヘモグロビンは4つのサブユニットを持つ球状のタンパク質で、それぞれのサブユニットには酸素と結合できる**ヘム基(heme group)**が含まれています。ヘム基は鉄イオン(Fe2+)と酸素が結合する部分です。
- 1つのヘモグロビン分子は最大4つの酸素分子を結合できます。
- **酸素飽和度(% saturation)**は、ヘモグロビンの全てのヘム基が酸素と結合している割合を示します。例えば、100%飽和は、全てのヘム基が酸素と結合している状態です。
- 酸素が結合しているヘモグロビンは酸化ヘモグロビン(oxyhemoglobin)、結合していないヘモグロビンは**脱酸素ヘモグロビン(deoxyhemoglobin)**と呼ばれます。
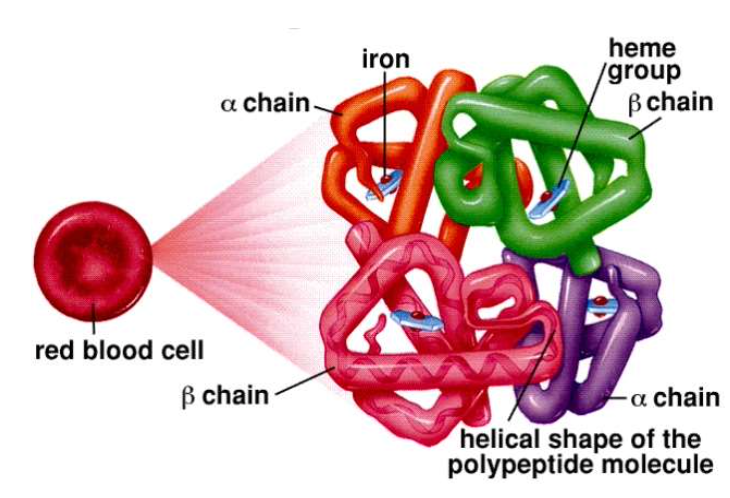
3. ヘモグロビンの異常型および変異型
- メトヘモグロビン(Methemoglobin)
- ヘム基の鉄イオンが**Fe3+(フェリック状態)**になってしまうと酸素と結合できません。これは、亜硝酸塩やサルファ剤(sulfonamides)によって酸化される場合などで見られます。
- また、**メトヘモグロビン還元酵素(methemoglobin reductase)**が先天的に欠損していると、この状態が持続します。
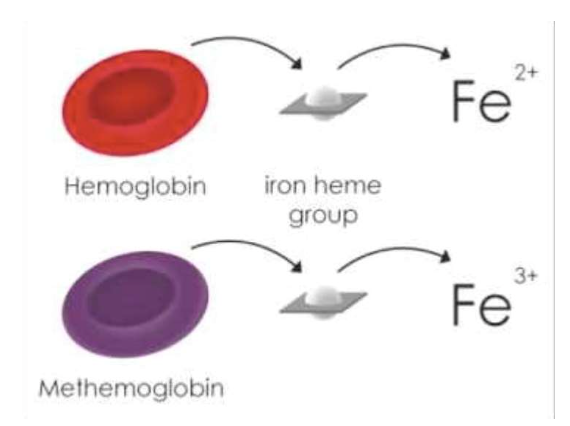
- ヘモグロビンF(Hemoglobin F)
- 胎児型ヘモグロビンであり、β鎖の代わりに**γ鎖(α2γ2)**を持ちます。母体から胎児への酸素移動を助けるため、ヘモグロビンA(成人型ヘモグロビン)よりも酸素親和性が高いです。
- 生後1年以内にヘモグロビンAに置き換わります。
- ヘモグロビンS(Hemoglobin S)
- **鎌状赤血球症(Sickle Cell Disease)**を引き起こす異常型ヘモグロビンです。α鎖は正常ですが、β鎖が変異しており、αA2βS2という構造を持ちます。
- 酸素が結合していない(脱酸素状態の)ヘモグロビンSは、赤血球内で鎌状の棒状構造を形成し、これが赤血球を鎌状に変形させます。これにより、赤血球が小血管を詰まらせて痛みを伴う鎌状赤血球症クリーゼを引き起こすことがあります。
- ヘモグロビンSは、ヘモグロビンAに比べて酸素親和性が低いです。
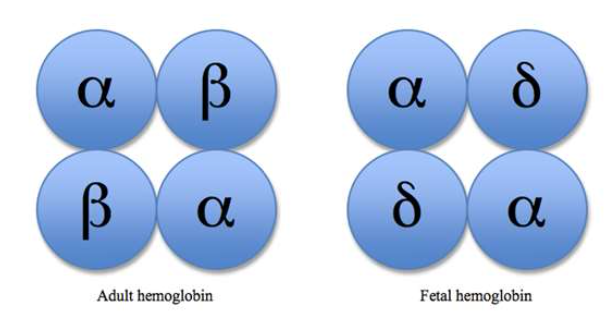
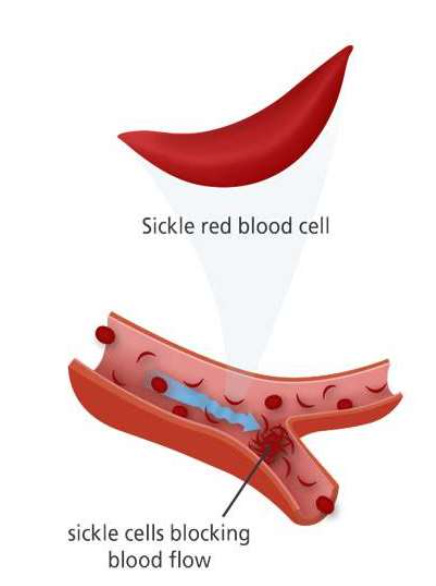
酸素の輸送:肺から体組織へ
酸素は肺から体の各組織へと輸送され、これには以下のプロセスが関与します。
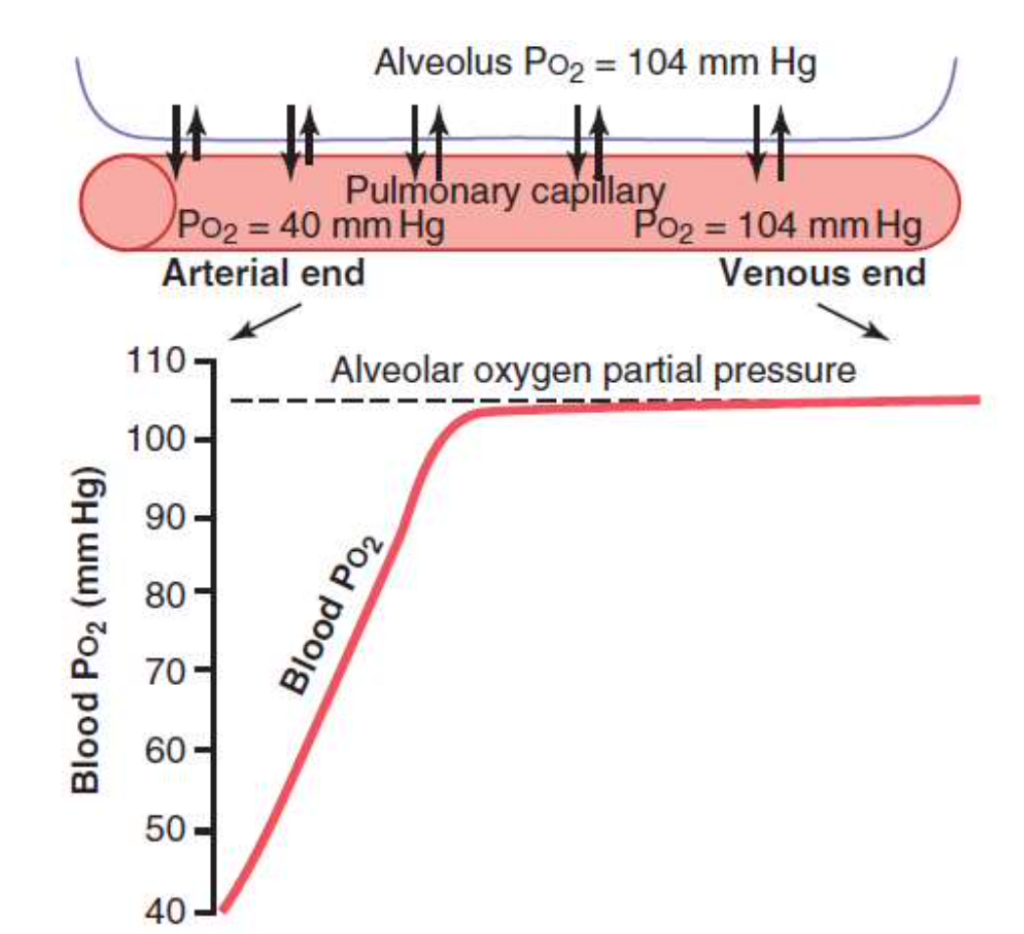
1. 肺胞から肺毛細血管への酸素の拡散
- 初期の圧力差: 肺胞内の酸素分圧(PAO2)が104 mm Hgであり、肺毛細血管内の酸素分圧(PaO2)が40 mm Hgであるため、酸素は肺胞から血液中へと拡散します。この圧力差は64 mm Hgです。
- 血液中の酸素分圧の上昇: 血液が毛細血管を通過するにつれて、酸素分圧は急速に上昇します。血液が毛細血管を三分の一移動すると、血液の酸素分圧は肺胞の空気の酸素分圧にほぼ達します。
- 運動中の変化: 運動中は酸素の拡散能力がほぼ三倍に増加します。これは、筋肉が必要とする酸素量が増えるためです。
2. 末梢毛細血管から組織液への酸素の拡散
- 組織液の酸素分圧: 組織液の酸素分圧(PO2)は平均的に40 mm Hgです。
- 大きな圧力差: 酸素は毛細血管から組織液へと急速に拡散します。毛細血管内の酸素分圧が40 mm Hgに近づきます。
- 組織毛細血管から静脈へ: 組織毛細血管を通過した血液の酸素分圧は40 mm Hgであり、静脈血がこの酸素分圧になります。
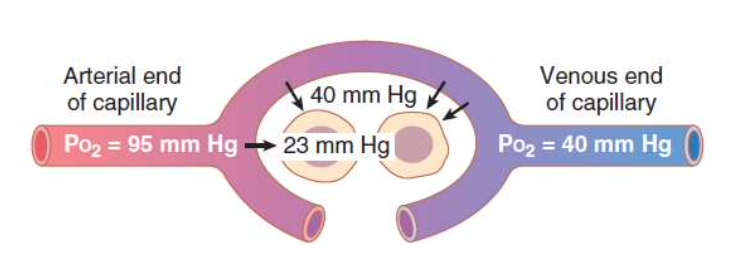
3. 末梢毛細血管から細胞内への酸素の拡散
- 細胞内の酸素分圧: 末梢組織内の酸素分圧(PO2)は毛細血管内よりも低いです。正常な細胞内の酸素分圧は5 mm Hgから40 mm Hgの間で、平均して23 mm Hgです。
- 必要な酸素圧: 酸素を使用する化学プロセスを支えるのに必要な酸素分圧は通常1から3 mm Hgであり、安全係数が大きいです。
ヘモグロビン-酸素解離曲線(Oxygen-Hemoglobin Dissociation Curve)
- グラフの形状: ヘモグロビンの酸素飽和度(% saturation)を酸素分圧(PO2)の関数としてプロットしたものです。曲線はS字形(シグモイド)です。
- 曲線の変化: 酸素分圧が0から約40 mm Hgまで上昇すると、ヘモグロビンの酸素飽和度が急激に上昇し、その後50 mm Hgから100 mm Hgの範囲では飽和度はほぼ一定になります。
酸素分圧とヘモグロビンの飽和度の例
- PO2 100 mm Hg: ヘモグロビンは100%飽和し、全てのヘム基が酸素と結合しています。
- PO2 40 mm Hg: ヘモグロビンは75%飽和し、平均して3つのヘム基が酸素と結合しています。
- PO2 25 mm Hg: ヘモグロビンは50%飽和し、50%飽和の酸素分圧をP50と呼びます。平均して2つのヘム基が酸素と結合しています。
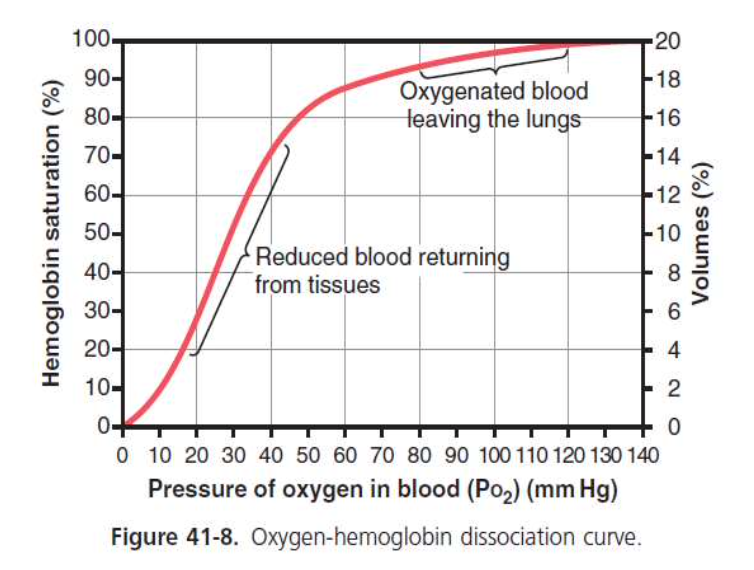
シグモイド形状の原因
- ポジティブコラボラティビティ: 最初の酸素分子が結合すると、次の酸素分子の結合に対する親和性が高まります。4番目の酸素分子の親和性が最も高くなります。
- 曲線の役割: 曲線の平坦な部分は肺での酸素の取り込みを、急な部分は組織での酸素の放出を容易にします。
呼吸器内でと末梢組織での酸素の動態
肺内で
- 肺胞内の酸素分圧: 100 mm Hg
- 肺毛細血管内の酸素分圧: 酸素が肺胞から血液中に拡散し、血液の酸素分圧も100 mm Hgになります。ヘモグロビンの酸素に対する親和性が高いため、酸素がしっかり結合し、酸素分圧が低く保たれ、拡散が効率的に行われます。
- 曲線のフラット部分: 酸素分圧が60から100 mm Hgの範囲では、曲線はほぼ平坦です。これにより、大気圧の変化に対してもヘモグロビンの酸素運搬能力は保たれます。
末梢組織で
- 酸素の拡散: 動脈血から細胞に酸素が拡散します。細胞が酸素を消費することで、組織内の酸素分圧が低く保たれます。
- ヘモグロビンの低親和性: 酸素分圧が低いとき、ヘモグロビンの酸素親和性が低くなり、酸素が組織に放出されやすくなります。
- 酸素の輸送量: 通常、動脈血は97%飽和で、組織での酸素飽和度は75%であり、約5 mLの酸素が100 mLの血液ごとに組織に輸送されます。
酸素の「バッファー」作用
- 「組織酸素バッファー」システム: 血液中のヘモグロビンは組織の酸素分圧を安定させる役割を果たします。組織が必要とする酸素量を確保しながら、酸素分圧を約40 mm Hgに保つことができます。
- 運動時の酸素供給: 激しい運動時には、酸素の需要が増加しますが、ヘモグロビンの解離曲線の急な傾斜と組織血流の増加により、酸素の供給が容易になります。少しの酸素分圧の低下で大量の酸素が放出されます。
大気中の酸素濃度の変化
- 高地や航空機: 高度が上がると肺胞酸素分圧が60 mm Hgまで低下しても、動脈血の酸素飽和度は89%で、正常値97%から8%低下するだけです。組織は依然として100 mLの血液ごとに約5 mLの酸素を取り除きます。
- 深海や高圧室: 高圧環境では、肺胞酸素分圧が500 mm Hgに達しても、ヘモグロビンの最大酸素飽和度は100%にしかなりません。追加の酸素は血液中にわずかに溶解します。
ヘモグロビン–酸素解離曲線の変化
ヘモグロビン–酸素解離曲線のシフトは、ヘモグロビンの酸素に対する親和性の変化を反映しています。この曲線のシフトは酸素の取り込みや放出に影響を与えます。以下に、シフトの種類とその原因を説明します。
右シフト(Right Shift)
右シフトは、ヘモグロビンの酸素に対する親和性が低下した場合に起こります。これにより、酸素の放出が促進されます。
- P50の増加: 酸素分圧が50%飽和の状態での値が増加します。
- 酸素の放出: 酸素が動脈血から組織へより容易に放出されます。
- 酸素飽和度の低下: どの酸素分圧においても、ヘモグロビンの酸素飽和度が低くなります。
右シフトの原因:
- CO2の増加やpHの低下(Bohr効果):
- CO2の増加やpHの低下はヘモグロビンの酸素親和性を低下させ、組織への酸素供給を促進します。例えば、運動中は筋肉がより多くのCO2を生成し、pHが低下し、酸素の供給が促進されます。
- 温度の上昇:
- 温度の上昇もヘモグロビンの酸素親和性を低下させ、酸素の組織への供給が容易になります。例えば、運動中の体温上昇がこれに該当します。
- 2,3-DPGの増加:
- 2,3-DPGはヘモグロビンのβ鎖に結合し、酸素の親和性を低下させます。慢性的な低酸素状態(例:高地に住む)では2,3-DPGの合成が増加し、酸素の放出が促進されます。
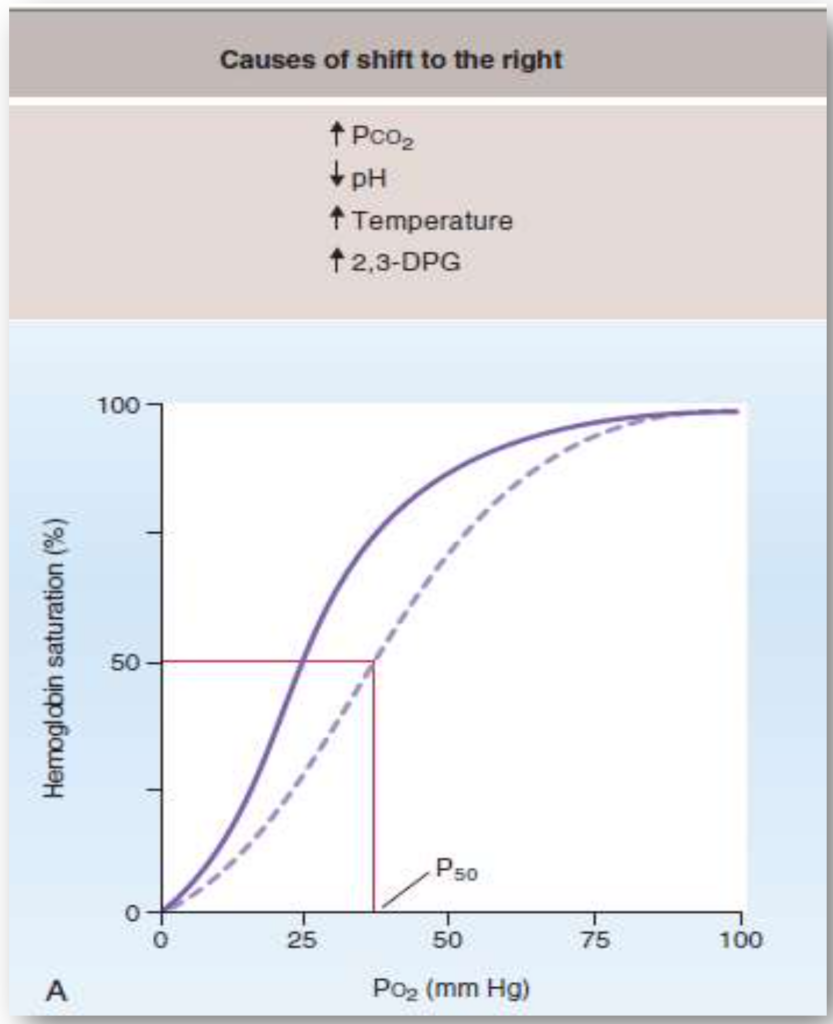
左シフト(Left Shift)
左シフトは、ヘモグロビンの酸素に対する親和性が増加した場合に起こります。これにより、酸素の放出が困難になります。
- P50の減少: 酸素分圧が50%飽和の状態での値が減少します。
- 酸素の放出困難: 酸素が動脈血から組織へ放出されにくくなります。
- 酸素飽和度の増加: どの酸素分圧においても、ヘモグロビンの酸素飽和度が高くなります。
左シフトの原因:
- CO2の減少:
- CO2が減少するとヘモグロビンの酸素親和性が増加し、酸素の放出が困難になります。
- pHの上昇:
- pHが上昇するとヘモグロビンの酸素親和性が増加します。
- 温度の低下:
- 温度が低下すると、ヘモグロビンの酸素親和性が増加します。
- 2,3-DPGの減少:
- 2,3-DPGの濃度が低下すると、ヘモグロビンの酸素親和性が増加し、酸素の放出が困難になります。
- HbF(胎児型ヘモグロビン):
- HbFは2,3-DPGとの結合が弱く、酸素の親和性が高いです。その結果、P50が低くなり、曲線は左にシフトします。
- 一酸化炭素(CO)中毒:
- COはヘモグロビンの酸素結合部位に競合的に結合し、酸素の親和性を200倍増加させます。これにより酸素の結合部位が占有され、残りの酸素の親和性が高まり、曲線が左にシフトします。
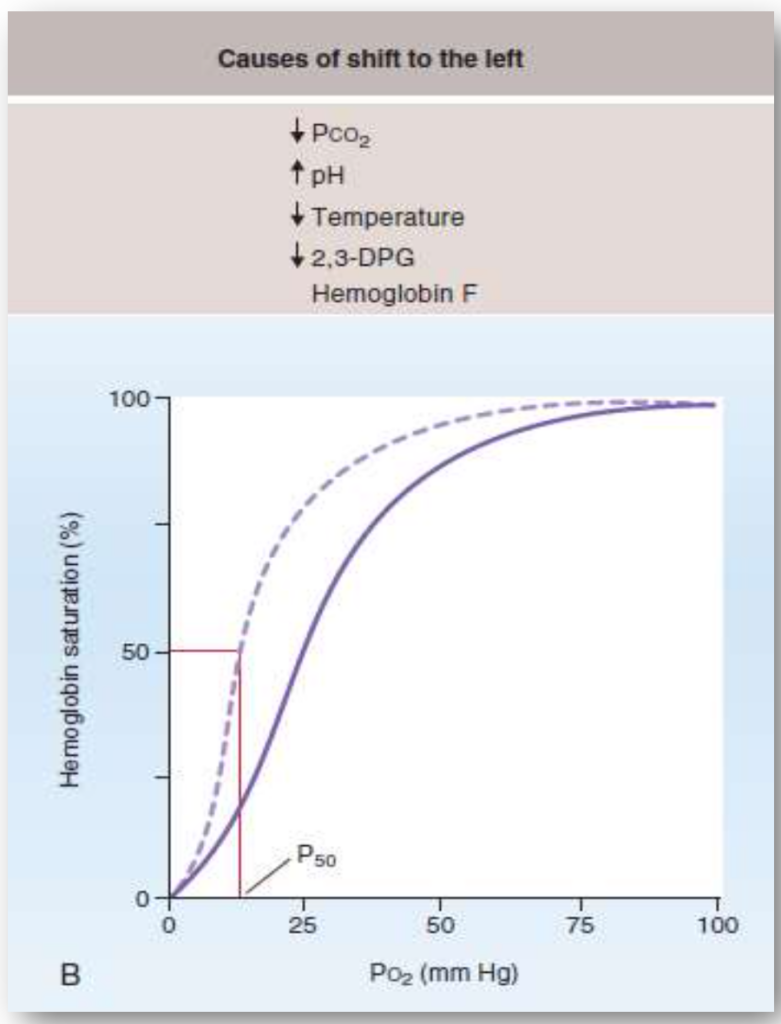
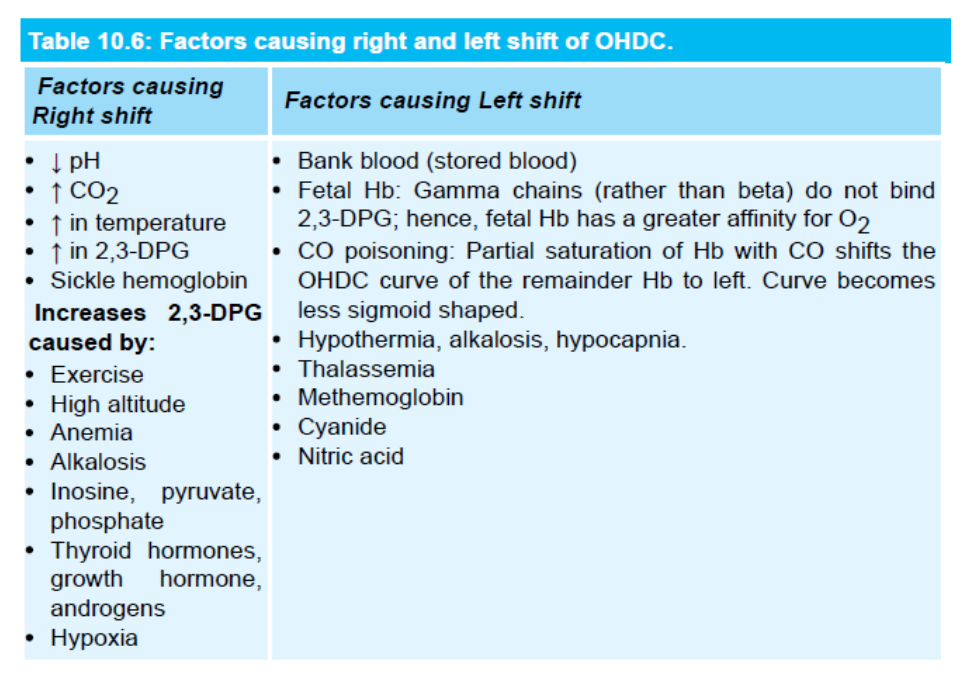
細胞内の酸素代謝への影響
酸素使用速度に対する細胞内酸素分圧の影響
- 酸素分圧が1 mm Hg以上: 細胞内の酸素使用速度は一定のADP濃度に対して一定になります。
- ADP濃度の変化: ADP濃度の変化に応じて酸素使用速度も変化します。ADP濃度が主な制限因子です。
- エネルギー消費の影響: 細胞内のエネルギー消費率が酸素使用速度を最終的に制御します。ATPからADPへの変換速度が関連しています。
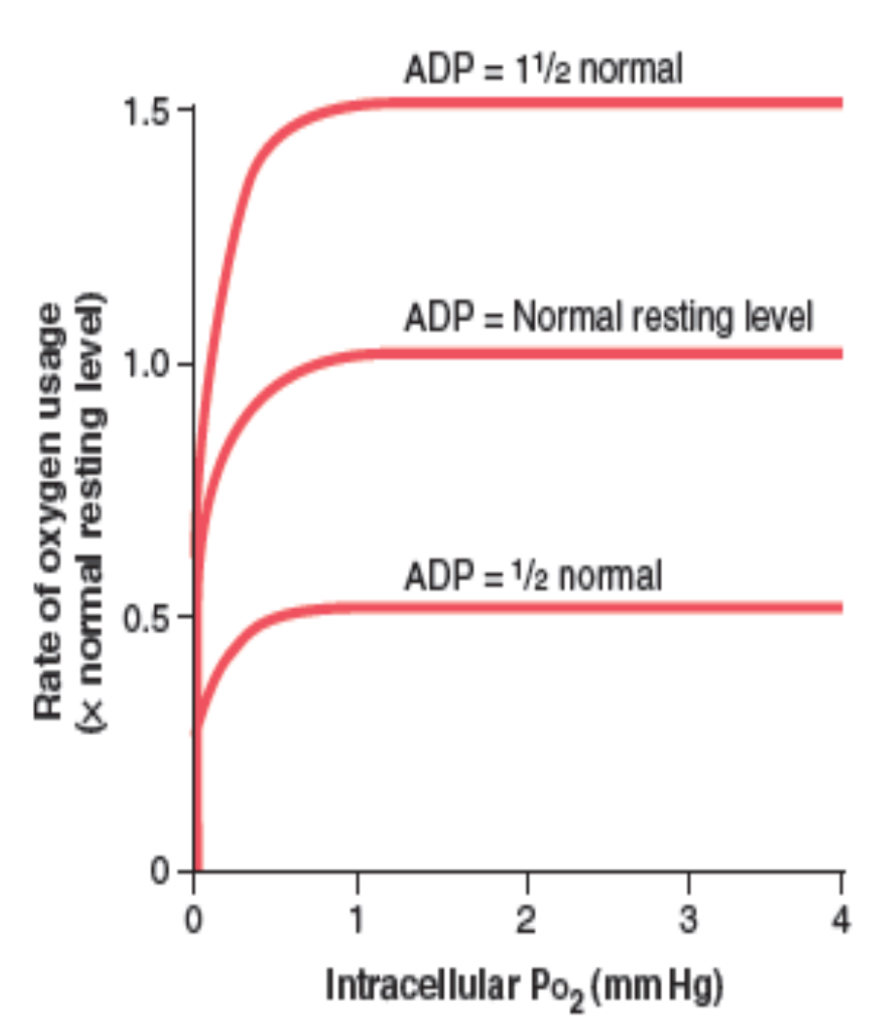
毛細血管から細胞への拡散距離の影響
- 拡散距離が長い場合: 細胞が毛細血管から遠くなると、酸素の拡散速度が低下し、細胞内の酸素分圧が臨界レベルを下回ることがあります。これにより、酸素使用が拡散に制限され、ADP濃度の変化に依存しなくなります。
- 病的状態: 通常、健康な状態ではこの状況は起こりません。
血流の影響
- 酸素供給量: 血液が1分間に供給する酸素量は、血液100 mLあたりの酸素量と血流速度によって決まります。
- 限界: 拡散に制限された状態や血流に制限された状態は長く続かず、細胞は必要な酸素量を受け取ることができなくなります。
二酸化炭素(CO2)の血液中での輸送
CO2の輸送
- CO2はO2よりもはるかに多く輸送されることができます。
- 血液中のCO2の量は、体液の酸塩基バランスにおいて重要です。
- 一般的に、血液の100 mLあたり約4 mLのCO2が組織から肺に運ばれます。
組織でのCO2の取り扱い
- CO2は、好気的代謝(aerobic metabolism)から生成されます。
- CO2は細胞膜を通って血液中に拡散します(単純拡散、simple diffusion)。
- CO2の分圧勾配(partial pressure gradient)によって駆動されます。
赤血球内での反応
- 赤血球内では、炭酸脱水酵素(carbonic anhydrase)がCO2の水和反応を触媒します。これは、CO2がH2Oと反応して炭酸(H2CO3)を形成する反応です。
- この反応はCO2の供給により右にシフトします。H2CO3は**H+とHCO3−**に解離します。
- H+は赤血球内に留まり、脱酸ヘモグロビン(deoxyhemoglobin)によって緩衝されます。
- HCO3−は血漿中に輸送され、クロライド(Cl−)と交換されます。この現象をクロライドシフト(chloride shift)と言います。
- HCO3−は、赤血球膜を通してCl−と交換され、バンド3タンパク質(band 3 protein、またはbicarbonate-chloride carrier protein)という陰イオン交換タンパク質によって肺に運ばれます。
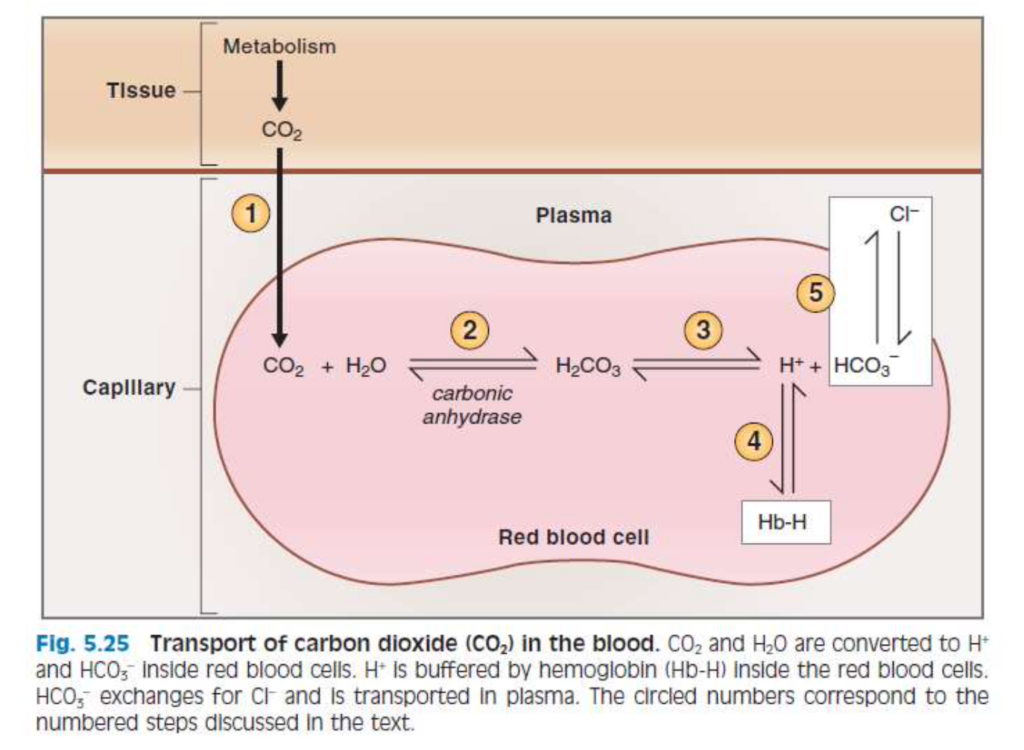
CO2の形態
- 溶解CO2(dissolved CO2):
- 血液中の一部のCO2は溶解した形で存在します。
- ヘンリーの法則(Henry’s law)により、CO2の濃度はCO2の分圧に比例します。
- CO2の溶解度は0.07 mL CO2/100 mL血液/mm Hgです。これは血液中のCO2の約7%に相当します。
- カルバミノヘモグロビン(carbaminohemoglobin、CO2Hgb):
- CO2はタンパク質の末端アミノ基に結合します。ヘモグロビン(hemoglobin)や血漿中のタンパク質に結合します。
- CO2はヘモグロビンのO2結合部位とは異なる部位に結合します。
- CO2がヘモグロビンに結合すると、O2の親和性が減少し、O2-ヘモグロビン解離曲線が右にシフトします(ボーア効果、Bohr effect)。
- HCO3−:
- 全CO2の90%以上を占めます。
- CO2とH2Oが反応してH2CO3を形成し、炭酸脱水酵素によって触媒されます。
- H2CO3はH+とHCO3−に解離します。
CO2解離曲線
- 血液中のCO2の総量がPCO2に依存する様子を示します。
- 正常なPCO2は動脈血で約40 mmHg、静脈血で約45 mmHgです。
- CO2の血液中の濃度は約50体積%です。CO2の正常な輸送では、4体積%だけが交換されます。
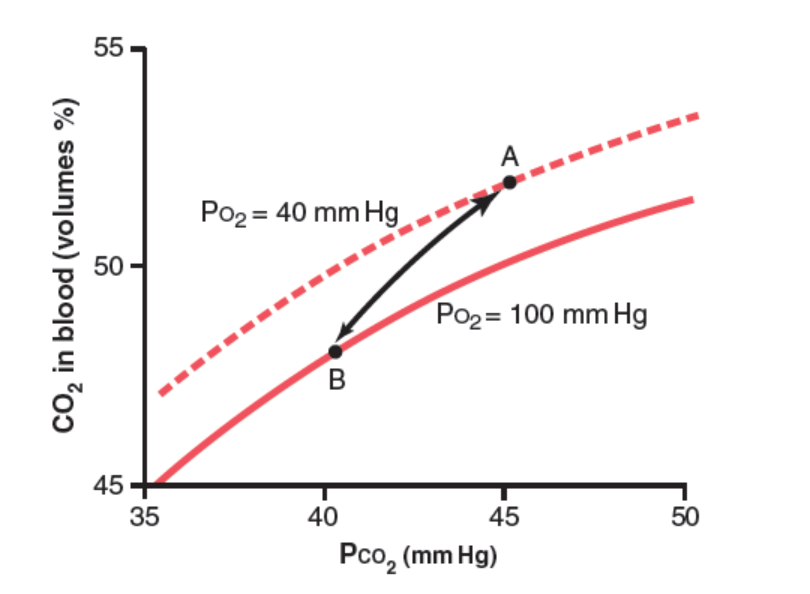
低酸素症(Hypoxia)
- 低酸素症(hypoxia): 組織レベルでのO2不足。
- 低酸素血症(hypoxemia): 動脈血中の酸素分圧(PaO2)の低下。
- 低酸素症の4つのカテゴリ:
- 低酸素性低酸素症(hypoxic hypoxia): 動脈血のPO2が低下します。
- 貧血性低酸素症(anemic hypoxia): 動脈PO2は正常ですが、O2を運ぶためのヘモグロビン量が減少します。
- 停滞性または虚血性低酸素症(stagnant or ischemic hypoxia): 血流が低く、O2が組織に適切に供給されません。
- 毒素性低酸素症(histotoxic hypoxia): 組織に十分なO2が供給されていますが、毒性物質の影響で細胞がO2を利用できません。
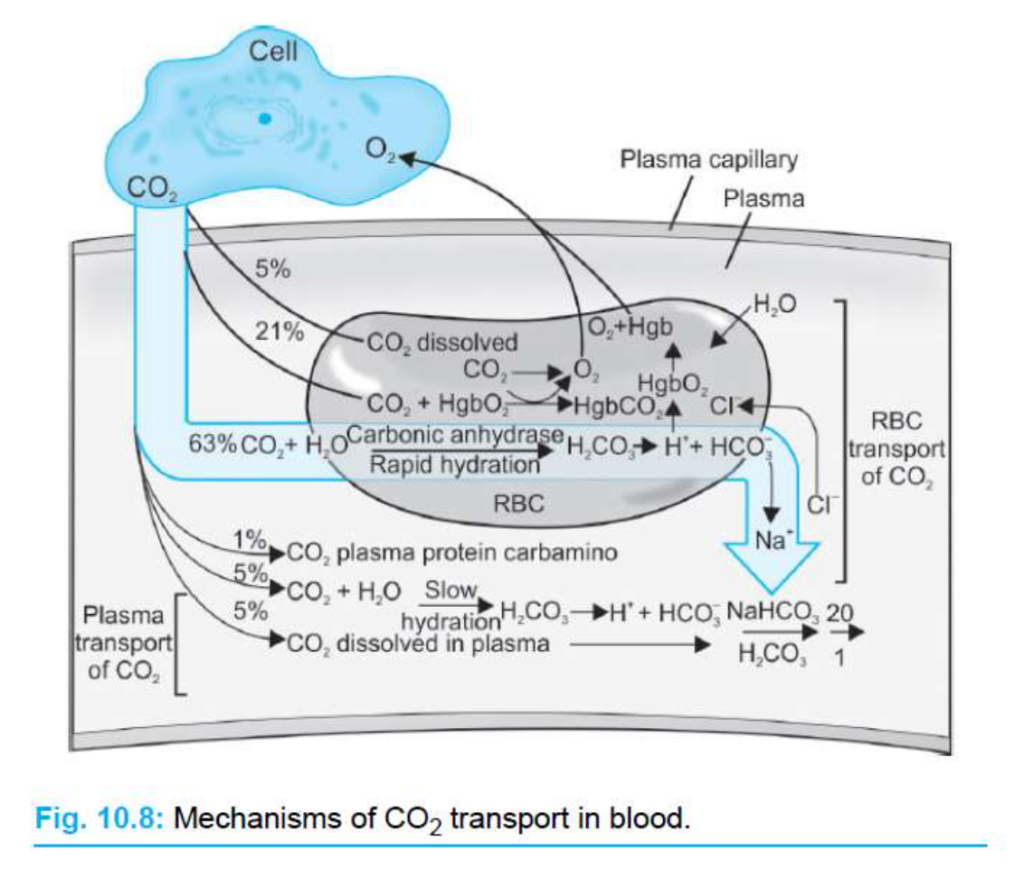
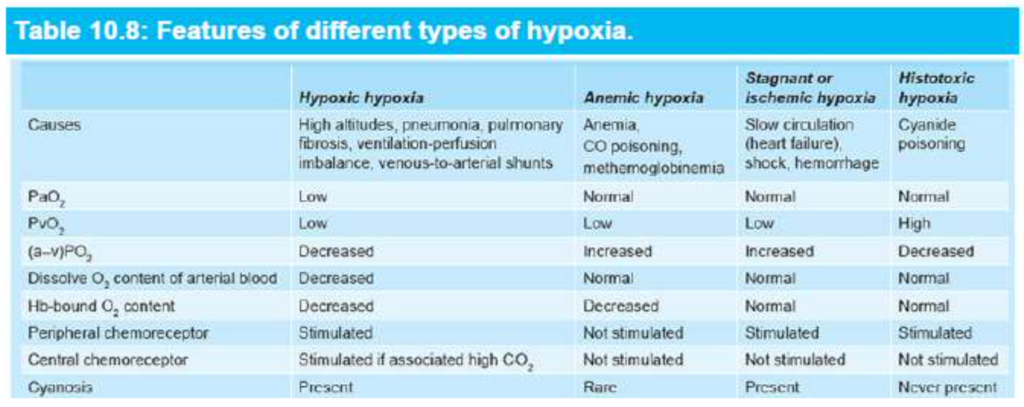
CHAPTER 40
Principles of Gas Exchange; Diffusion of Oxygen and Carbon Dioxide Through the Respiratory Membrane
After the alveoli are ventilated with fresh air, the next step in respiration is diffusion of oxygen (O2) from the alveoli into the pulmonary blood and diffusion of carbon diox- ide (CO2) in the opposite direction, out of the blood into the alveoli. The process of diffusion is simply the random motion of molecules in all directions through the respira- tory membrane and adjacent fluids. However, in respira- tory physiology, we are concerned not only with the basic mechanism by which diffusion occurs but also with the rate at which it occurs, which is a much more complex issue, requiring a deeper understanding of the physics of diffusion and gas exchange.
Physics of Gas Diffusion and Gas Partial Pressures
Molecular Basis of Gas Diffusion
All the gases of concern in respiratory physiology are sim- ple molecules that are free to move among one another by diffusion. This is also true of gases dissolved in the fluids and tissues of the body.
For diffusion to occur, there must be a source of energy. This source of energy is provided by the kinetic motion of the molecules. Except at absolute zero temperature, all molecules of all matter are continually undergoing motion. For free molecules that are not physically attached to oth- ers, this means linear movement at high velocity until they strike other molecules. They then bounce away in new di- rections and continue moving until they strike other mol- ecules again. In this way, the molecules move rapidly and randomly among one another.
Net Diffusion of a Gas in One Direction—Effect of a Concentration Gradient. If a gas chamber or solution has a high concentration of a particular gas at one end of the cham- ber and a low concentration at the other end, as shown in Figure 40-1, net diffusion of the gas will occur from the high- concentration area toward the low-concentration area. The reason is obvious. There are far more molecules at end A of the chamber to diffuse toward end B than there are molecules to diffuse in the opposite direction. Therefore, the rates of diffu- sion in each of the two directions are proportionately different, as demonstrated by the lengths of the arrows in the figure.
Gas Pressures in a Mixture of Gases—Partial Pressures of Individual Gases
Pressure is caused by multiple impacts of moving mole- cules against a surface. Therefore, the pressure of a gas act-
ing on the surfaces of the respiratory passages and alveoli is proportional to the summated force of impact of all the molecules of that gas striking the surface at any given in- stant. This means that the pressure is directly proportional to the concentration of the gas molecules.
In respiratory physiology, one deals with mixtures of gases, mainly oxygen, nitrogen, and carbon dioxide. The rate of diffusion of each of these gases is directly proportional to the pressure caused by that gas alone, which is called the partial pressure of that gas. The concept of partial pressure can be explained as follows.
Consider air, which has an approximate composition of 79% nitrogen and 21% oxygen. The total pressure of this mixture at sea level averages 760 mm Hg. It is clear from the preceding description of the molecular basis of pres- sure that each gas contributes to the total pressure in direct proportion to its concentration. Therefore, 79% of the 760 mm Hg is caused by nitrogen (600 mm Hg) and 21% by O2 (160 mm Hg). Thus, the partial pressure of nitrogen in the mixture is 600 mm Hg, and the partial pressure of O2 is 160 mm Hg; the total pressure is 760 mm Hg, the sum of the individual partial pressures. The partial pressures of individual gases in a mixture are designated by the symbols Po2, Pco2, Pn2, Phe, and so forth.
Pressures of Gases Dissolved in Water and Tissues
Gases dissolved in water or in body tissues also exert pres- sure because the dissolved gas molecules are moving ran- domly and have kinetic energy. Furthermore, when the gas dissolved in fluid encounters a surface, such as the mem- brane of a cell, it exerts its own partial pressure in the same way as a gas in the gas phase. The partial pressures of the separate dissolved gases are designated the same as the par- tial pressures in the gas state—that is, Po2, Pco2, Pn2, Phe, and so forth.
Factors That Determine Partial Pressure of a Gas Dissolved in a Fluid. The partial pressure of a gas in a so- lution is determined not only by its concentration but also by the solubility coefficient of the gas. That is, some types of molecules, especially CO2, are physically or chemically attracted to water molecules, whereas other types of mol- ecules are repelled. When molecules are attracted, far more of them can be dissolved without building up excess par- tial pressure within the solution. Conversely, in the case of molecules that are repelled, high partial pressure will devel- op with fewer dissolved molecules. These relationships areexpressed by the following formula, which is Henry’s law: Partial pressure = Concentration of dissolved gas
Solubility coefficient
When partial pressure is expressed in atmospheres (1 atmosphere [1 atm] pressure equals 760 mm Hg) and con- centration is expressed in volume of gas dissolved in each volume of water, the solubility coefficients for important respiratory gases at body temperature are the following:
Oxygen:
Carbon dioxide:
Carbon monoxide:
Nitrogen:
Helium:
0.024
0.57
0.018
0.012
0.008
From this list, one can see that CO2 is more than 20 times as soluble as O2. Therefore, the partial pressure of CO2 for a given concentration is less than one-twentieth (5%) of that exerted by O2.
Diffusion of Gases Between Gas Phase in Alveoli and Dissolved Phase in Pulmonary Blood. The partial pressure of each gas in the alveolar respiratory gas mixture tends to force molecules of that gas into solution in the blood of the alveo- lar capillaries. Conversely, the molecules of the same gas that are already dissolved in the blood are bouncing randomly in the fluid of the blood, and some of these bouncing molecules escape back into the alveoli. The rate at which they escape is directly proportional to their partial pressure in the blood.
But, in which direction will net diffusion of the gas oc- cur? The answer is that net diffusion is determined by the difference between the two partial pressures. If the partial pressure is greater in the gas phase in the alveoli, as is nor- mally true for oxygen, then more molecules will diffuse into the blood than in the other direction. Alternatively, if the partial pressure of the gas is greater in the dissolved state in the blood, which is normally true for CO2, then net diffu- sion will occur toward the gas phase in the alveoli.
Vapor Pressure of Water
When nonhumidified air is breathed into the respiratory passageways, water immediately evaporates from the surfac- es of these passages and humidifies the air. This results from the fact that water molecules, like different dissolved gas molecules, are continually escaping from the water surface into the gas phase. The partial pressure that water molecules exert to escape through the surface is called the vapor pres- sure of the water. At normal body temperature, 37°C (98.6°F), this vapor pressure is 47 mm Hg. Therefore, once the gas mixture has become fully humidified—that is, once it is in equilibrium with the water—the partial pressure of the water vapor in the gas mixture is 47 mm Hg. This partial pressure, like the other partial pressures, is designated as Ph2o.
The vapor pressure of water depends entirely on the tem- perature of the water. The higher the temperature, the great- er the kinetic activity of the molecules and, therefore, the greater the likelihood that the water molecules will escape from the surface of the water into the gas phase. For example, the water vapor pressure at 0°C is 5 mm Hg, and at 100°C it is 760 mm Hg. The most important value to remember is the vapor pressure at body temperature, 47 mm Hg. This value appears in many of our subsequent discussions.
Pressure Difference Causes Net Diffusion of Gases Through Fluids
From the preceding discussion, it is clear that when the par- tial pressure of a gas is greater in one area than in another area, there will be net diffusion from the high-pressure area toward the low-pressure area. For example, returning to Figure 40-1, one can readily see that the molecules in the area of high pressure, because of their greater number, have a greater chance of moving randomly into the area of low pres- sure than do molecules attempting to go in the other direc- tion. However, some molecules do bounce randomly from the low-pressure area toward the high-pressure area. There- fore, the net diffusion of gas from the area of high pressure to the area of low pressure is equal to the number of mol- ecules bouncing in this forward direction minus the number bouncing in the opposite direction, which is proportional to the gas partial pressure difference between the two areas, called simply the pressure difference for causing diffusion.
Quantifying Net Rate of Diffusion in Fluids. In addition to the pressure difference, several other factors affect the rate of gas diffusion in a fluid: (1) the solubility of the gas in the fluid; (2) the cross-sectional area of the fluid; (3) the dis- tance through which the gas must diffuse; (4) the molecular weight of the gas; and (5) the temperature of the fluid. In the body, the temperature remains reasonably constant and usually need not be considered.
The greater the solubility of the gas, the greater the num- ber of molecules available to diffuse for any given partial pressure difference. The greater the cross-sectional area of the diffusion pathway, the greater the total number of mol- ecules that diffuse. Conversely, the greater the distance the molecules must diffuse, the longer it will take the molecules to diffuse the entire distance. Finally, the greater the veloc- ity of kinetic movement of the molecules, which is inversely proportional to the square root of the molecular weight, the greater the rate of diffusion of the gas. All these factors can be expressed in a single formula, as follows:
ΔP×A×S D∝ d×√MW
in which D is the diffusion rate, ΔP is the partial pres- sure difference between the two ends of the diffusion path- way, A is the cross-sectional area of the pathway, S is the solubility of the gas, d is the distance of diffusion, and MW is the molecular weight of the gas.
It is obvious from this formula that the characteristics of the gas determine two factors of the formula—solubility and molecular weight. Together, these two factors deter- mine the diffusion coefficient of the gas, which is propor- tional to S/√MW; that is, the relative rates at which differ- ent gases at the same partial pressure levels will diffuse are proportional to their diffusion coefficients. Assuming that the diffusion coefficient for O2 is 1, the relative diffusion coefficients for different gases of respiratory importance in the body fluids are as follows:
Oxygen: 1.0
Carbon dioxide: 20.3
Carbon monoxide: 0.81
Nitrogen: 0.53
Helium: 0.95
Diffusion of Gases Through Tissues
The gases that are of respiratory importance are all highly soluble in lipids and, consequently, are highly soluble in cell membranes. Because of this, the major limitation to move- ment of gases in tissues is the rate at which the gases can diffuse through the tissue water instead of through the cell membranes. Therefore, diffusion of gases through tissues, in- cluding through the respiratory membrane, is almost equal to the diffusion of gases in water, as given in the preceding list.
COMPOSITIONS OF ALVEOLAR AIR AND ATMOSPHERIC AIR ARE DIFFERENT
Alveolar air does not have the same concentrations of gases as atmospheric air (Table 40-1). There are several reasons for the differences. First, alveolar air is only par- tially replaced by atmospheric air with each breath. Sec- ond, O2 is constantly being absorbed into the pulmonary blood from the alveolar air. Third, CO2 is constantly dif- fusing from the pulmonary blood into the alveoli. And fourth, dry atmospheric air that enters the respiratory passages is humidified even before it reaches the alveoli.
Air Is Humidified in the Respiratory Passages
Table 40-1 shows that atmospheric air is composed almost entirely of nitrogen and O2; it normally contains almost no CO2 and little water vapor. However, as soon as the atmo- spheric air enters the respiratory passages, it is exposed to the fluids that cover the respiratory surfaces. Even before the air enters the alveoli, it becomes almost totally humidified.
The partial pressure of water vapor at a normal body temperature of 37°C is 47 mm Hg, which is therefore the partial pressure of water vapor in the alveolar air. Because the total pressure in the alveoli cannot rise to more than the atmospheric pressure (760 mm Hg at sea level), this water vapor simply dilutes all the other gases in the inspired air. Table 40-1 also shows that humidification of the air dilutes the oxygen partial pressure at sea level from an average of 159 mm Hg in atmospheric air to 149 mm Hg in the humidified air, and it dilutes the nitrogen partial pressure from 597 to 563 mm Hg.
Alveolar Air Is Slowly Renewed by Atmospheric Air
In Chapter 38, we pointed out that the average functional residual capacity of the lungs (the volume of air remain- ing in the lungs at the end of normal expiration) measures about 2300 ml in men. Yet only 350 ml of new air is brought into the alveoli with each normal inspiration, and this same amount of old alveolar air is expired. Therefore, the volume of alveolar air replaced by new atmospheric air with each breath is only one-seventh of the total, so multiple breaths are required to exchange most of the alveolar air. Figure 40-2 shows this slow rate of renewal of the alveolar air. In the first alveolus of the figure, excess gas is present in the alveoli, but note that even at the end of 16 breaths, the excess gas still has not been completely removed from the alveoli.
Figure 40-3 demonstrates graphically the rate at which excess gas in the alveoli is normally removed, showing that with normal alveolar ventilation, about half the gas is removed in 17 seconds. When a person’s rate of alveolar ventilation is only half-normal, half of the gas is removed in 34 seconds, and when the rate of ventilation is twice normal, half is removed in about 8 seconds.
Slow Replacement of Alveolar Air Helps Stabilize Res- piratory Control. The slow replacement of alveolar air is of particular importance in preventing sudden changes in gas concentrations in the blood. This makes the respira- tory control mechanism much more stable than it would be otherwise, and it helps prevent excessive increases and decreases in tissue oxygenation, tissue CO2 concentration, and tissue pH when respiration is temporarily interrupted.
Oxygen Concentration and Partial Pressure in Alveoli
Oxygen is continually being absorbed from the alveoli into the blood of the lungs, and new O2 is continually being breathed into the alveoli from the atmosphere. The more rapidly O2 is absorbed, the lower its concentration in the alveoli becomes; conversely, the more rapidly new O2 is breathed into the alveoli from the atmosphere, the higher its concentration becomes. Therefore, O2 concentration in the alveoli, as well as its partial pressure, is controlled by the following: (1) the rate of absorption of O2 into the blood; and (2) the rate of entry of new O2 into the lungs by the ventilatory process.
Figure 40-4 shows the effect of alveolar ventilation and rate of O2 absorption into the blood on the alveolar Po2. One curve represents O2 absorption at a rate of 250 ml/min, and the other curve represents a rate of 1000 ml/ min. At a normal ventilatory rate of 4.2 L/min and an O2 consumption of 250 ml/min, the normal operating point in Figure 40-4 is point A. The figure also shows that when 1000 ml of O2 is being absorbed each minute, as during moderate exercise, the rate of alveolar ventilation must increase fourfold to maintain the alveolar Po2 at the nor- mal value of 104 mm Hg.
Another effect shown in Figure 40-4 is that even an extreme increase in alveolar ventilation can never increase the alveolar Po2 above 149 mm Hg as long as the person is breathing normal atmospheric air at sea level pressure, because 149 mm Hg is the maximum Po2 in humidified air at this pressure. If the person breathes gases that con- tain partial pressures of O2 higher than 149 mm Hg, the alveolar Po2 can approach these higher pressures at high rates of ventilation.
CO2 Concentration and Partial Pressure in Alveoli
Carbon dioxide is continually formed in the body and then carried in the blood to the alveoli; it is continually removed from the alveoli by ventilation. Figure 40-5 shows the effects on the alveolar partial pressure of Pco2 of both alveolar ventilation and two rates of CO2 excre- tion, 200 and 800 ml/min. One curve represents a normal rate of CO2 excretion of 200 ml/min. At the normal rate of alveolar ventilation of 4.2 L/min, the operating point for alveolar Pco2 is at point A in Figure 40-5 (i.e., 40 mm Hg). Two other facts are also evident from Figure 40-5. First, the alveolar Pco2 increases directly in proportion to the rate of CO2 excretion, as represented by the fourfold elevation of the curve (when 800 ml of CO2 are excreted per minute). Second, the alveolar Pco2 decreases in inverse proportion to alveolar ventilation. Therefore, the concentrations and partial pressures of both O2 and CO2 in the alveoli are determined by the rates of absorption or excretion of the two gases and by the amount of alveolar ventilation.
Expired Air Is a Combination of Dead Space Air and Alveolar Air
The overall composition of expired air is determined by the following: (1) the amount of the expired air that is dead space air; and (2) the amount that is alveolar air. Figure 40-6 shows the progressive changes in O2 and CO2 partial pressures in the expired air during the course of expiration. The first portion of this air, the dead space air from the res- piratory passageways, is typical humidified air, as shown in Table 40-1. Then, progressively more and more alveo- lar air becomes mixed with the dead space air until all the dead space air has finally been washed out, and nothing but alveolar air is expired at the end of expiration. Therefore, the method of collecting alveolar air for study is simply to collect a sample of the last portion of the expired air after forceful expiration has removed all the dead space air. Normal expired air, containing both dead space air and alveolar air, has gas concentrations and partial pressures ap- proximately as shown in Table 40-1 (i.e., concentrations be- tween those of alveolar air and humidified atmospheric air). DIFFUSION OF GASES THROUGH THE RESPIRATORY MEMBRANE
Respiratory Unit. Figure 40-7 shows the respira- tory unit (also called respiratory lobule), which is composed of a respiratory bronchiole, alveolar ducts, atria, and alveoli. There are about 300 mil- lion alveoli in the two lungs, and each alveolus has an average diameter of about 0.2 millimeter. The alveolar walls are extremely thin, and between the alveoli is an almost solid network of interconnect- ing capillaries, shown in Figure 40-8. Because of the extensiveness of the capillary plexus, the flow of blood in the alveolar wall has been described as a sheet of flowing blood. Thus, it is obvious that the alveolar gases are in very close proximity to the blood of the pulmonary capillaries. Furthermore, gas exchange between the alveolar air and pulmo- nary blood occurs through the membranes of all the terminal portions of the lungs, not merely in the al- veoli. All these membranes are collectively known as the respiratory membrane, also called the pulmo- nary membrane.
Respiratory Membrane. Figure 40-9 shows the ultra- structure of the respiratory membrane drawn in cross section on the left and a red blood cell on the right. It also shows diffusion of O2 from the alveolus into the red blood cell and diffusion of CO2 in the opposite direc- tion. Note the following different layers of the respiratory membrane:
1. A layer of fluid containing surfactant that lines the al- veolus and reduces the surface tension of alveolar fluid
A thin interstitial space between the alveolar epi- thelium and capillary membrane
The alveolar epithelium, composed of thin epithelial cells
An epithelial basement membrane
5. A capillary basement membrane that in many places fuses with the alveolar epithelial basement membrane
6. The capillary endothelial membrane
Despite the large number of layers, the overall thick- ness of the respiratory membrane in some areas is as little as 0.2 micrometer and averages about 0.6 microm- eter, except where there are cell nuclei. From histological studies, it has been estimated that the total surface area of the respiratory membrane is about 70 square meters in healthy men, which is equivalent to the floor area of a 25 × 30-foot room. The total quantity of blood in the capillar- ies of the lungs at any given instant is 60 to 140 ml. Now, imagine this small amount of blood spread over the entire surface of a 25 × 30-foot floor, and it is easy to understand the rapidity of the respiratory exchange of O2 and CO2.
The average diameter of the pulmonary capillaries is only about 5 micrometers, which means that red blood cells must squeeze through them. The red blood cell membrane usually touches the capillary wall, so O2 and CO2 need not pass through significant amounts of plasma as they diffuse between the alveolus and red blood cell. This, too, increases the rapidity of diffusion.
Factors Affecting Rate of Gas Diffusion Through the Respiratory Membrane
Referring to the earlier discussion of diffusion of gases in water, one can apply the same principles to diffusion of gases through the respiratory membrane. Thus, the fac- tors that determine how rapidly a gas will pass through the membrane are the following: (1) the thickness of the membrane; (2) the surface area of the membrane; (3) the diffusion coefficient of the gas in the substance of the membrane; and (4) the partial pressure difference of the gas between the two sides of the membrane.
The thickness of the respiratory membrane occasionally increases—for example, as a result of edema fluid in the interstitial space of the membrane and in the alveoli—so the respiratory gases must then diffuse not only through the membrane but also through this fluid. Also, some pul- monary diseases cause fibrosis of the lungs, which can increase the thickness of some portions of the respira- tory membrane. Because the rate of diffusion through the membrane is inversely proportional to the thickness of the membrane, any factor that increases the thickness to more than two to three times normal can interfere significantly with normal respiratory exchange of gases.
The surface area of the respiratory membrane can be greatly decreased by many conditions. For example, removal of an entire lung decreases the total surface area to half-normal. Also, in emphysema, many of the alveoli coalesce, with dissolution of many alveolar walls. There- fore, the new alveolar chambers are much larger than the original alveoli, but the total surface area of the respira- tory membrane is often decreased as much as fivefold because of loss of the alveolar walls. When the total sur- face area is decreased to about one-third to one-fourth normal, exchange of gases through the membrane is substantially impeded, even under resting conditions, and during competitive sports and other strenuous exercise, even the slightest decrease in surface area of the lungs can be a serious detriment to respiratory exchange of gases.
The diffusion coefficient for transfer of each gas through the respiratory membrane depends on the gas’s solubility in the membrane and, inversely, on the square root of the gas’s molecular weight. The rate of diffusion in the respira- tory membrane is almost exactly the same as that in water, for reasons explained earlier. Therefore, for a given pres- sure difference, CO2 diffuses about 20 times as rapidly as O2. Oxygen diffuses about twice as rapidly as nitrogen.
The pressure difference across the respiratory membrane is the difference between the partial pressure of the gas in the alveoli and the partial pressure of the gas in the pul- monary capillary blood. Therefore, the difference between these two pressures is a measure of the net tendency for the gas molecules to move through the membrane.
When the partial pressure of a gas in the alveoli is greater than the pressure of the gas in the blood, as is true for O2, net diffusion from the alveoli into the blood occurs. When the pressure of the gas in the blood is greater than the partial pressure in the alveoli, as is true for CO2, net diffusion from the blood into the alveoli occurs.
Diffusing Capacity of the Respiratory Membrane
The ability of the respiratory membrane to exchange a gas between the alveoli and pulmonary blood is expressed in quantitative terms by the respiratory membrane’s diffusing capacity, which is defined as the volume of a gas that will diffuse through the membrane each minute for a partial pressure difference of 1 mm Hg. All the factors discussed earlier that affect diffusion through the respiratory mem- brane can affect this diffusing capacity.
Diffusing Capacity for Oxygen. In the average young man, the diffusing capacity for O2 under resting conditions averages 21 ml/min per mm Hg. In functional terms, what does this mean? The mean O2 pressure difference across the respiratory membrane during normal quiet breathing is about 11 mm Hg. Multiplying this pressure by the diffusing capacity (11 × 21) gives a total of about 230 ml of oxygen diffusing through the respiratory membrane each minute, which is equal to the rate at which the resting body uses O2.
Increased Oxygen Diffusing Capacity During Exercise.
During strenuous exercise or other conditions that greatly increase pulmonary blood flow and alveolar ventilation, the diffusing capacity for O2 increases to about three times the diffusing capacity under resting conditions. This increase is caused by several factors, including the following: (1) opening up of many previously dormant pulmonary capil- laries or extra dilation of already open capillaries, thereby increasing the surface area of the blood into which the O2 can diffuse; and (2) a better match between the ventilation of the alveoli and perfusion of the alveolar capillaries with blood, called the ventilation-perfusion ratio, explained later in this chapter. Therefore, during exercise, oxygenation of the blood is increased not only by increased alveolar venti- lation but also by greater diffusing capacity of the respira- tory membrane for transporting O2 into the blood.
Diffusing Capacity for Carbon Dioxide. The diffusing capacity for CO2 has never been measured because CO2 diffuses through the respiratory membrane so rapidly that the average Pco2 in the pulmonary blood is not very dif- ferent from the Pco2 in the alveoli—the average difference is less than 1 mm Hg. With currently available techniques, this difference is too small to be measured.
Nevertheless, measurements of diffusion of other gases have shown that the diffusing capacity varies directly with the diffusion coefficient of the particular gas. Because the diffusion coefficient of CO2 is slightly more than 20 times that of O2, one would expect a diffusing capacity for CO2 under resting conditions of about 400 to 450 ml/min per mm Hg and during exercise of about 1200 to 1300 ml/ min per mm Hg. Figure 40-10 compares the measured or calculated diffusing capacities of carbon monoxide, O2, and CO2 at rest and during exercise, showing the extreme diffusing capacity of CO2 and the effect of exercise on the diffusing capacity of each of these gases.
Measurement of Diffusing Capacity—Carbon Monox- ide Method. The O2 diffusing capacity can be calculated from measurements of the following: (1) alveolar Po2; (2) Po2 in the pulmonary capillary blood; and (3) the rate of O2 uptake by the blood. However, measuring the Po2 in the pulmonary capillary blood is so difficult and imprecise that it is not practical to measure oxygen diffusing capacity by such a direct procedure, except on an experimental basis.
To obviate the difficulties encountered in measuring ox- ygen diffusing capacity directly, physiologists usually meas- ure carbon monoxide (CO) diffusing capacity instead and then calculate the O2 diffusing capacity from this. The prin- ciple of the CO method is as follows. A small amount of CO is breathed into the alveoli, and the partial pressure of the CO in the alveoli is measured from appropriate alveolar air samples. The CO pressure in the blood is essentially zero because hemoglobin combines with this gas so rapidly that its pressure never has time to build up. Therefore, the pres- sure difference of CO across the respiratory membrane is equal to its partial pressure in the alveolar air sample. Then, by measuring the volume of CO absorbed in a short period and dividing this by the alveolar CO partial pressure, one can determine the CO diffusing capacity accurately.
To convert CO diffusing capacity to O2 diffusing capac- ity, the value is multiplied by a factor of 1.23 because the diffusion coefficient for O2 is 1.23 times that for CO. Thus, the average diffusing capacity for CO in healthy young men at rest is 17 ml/min per mm Hg, and the diffusing capacity for O2 is 1.23 times this, or 21 ml/min per mm Hg.
Effect of Ventilation-Perfusion Ratio on Alveolar Gas Concentration
Earlier in this chapter, we learned that two factors deter- mine the Po2 and Pco2 in the alveoli: (1) the rate of alveo- lar ventilation; and (2) the rate of transfer of O2 and CO2 through the respiratory membrane. This discussion made the assumption that all the alveoli are ventilated equally, and that blood flow through the alveolar capillaries is the same for each alveolus. However, even normally to some extent, and especially in many lung diseases, some areas of the lungs are well ventilated but have almost no blood flow, whereas other areas may have excellent blood flow but little or no ventilation. In either of these conditions, gas exchange through the respiratory membrane is seri- ously impaired, and the person may suffer severe respira- tory distress, despite normal total ventilation and normal total pulmonary blood flow, but with the ventilation and blood flow going to different parts of the lungs. Therefore, a highly quantitative concept has been developed to help us understand respiratory exchange when there is imbalance between alveolar ventilation and alveolar blood flow. This concept is called the ventilation-perfusion ratio.
In quantitative terms, the ventilation-perfusion ratio is ex- pressed as V ̇ A/Q ̇ . When ̇V ̇ A (alveolar ventilation) is normal for a given alveolus, and Q (blood flow) is also normal for the same alveolus, the ventilation-perfusion ratio (V ̇ A/Q ̇ ) is also said to be normal. When the ventilation (V ̇ A) is zero, yet there isstillperfusion(Q ̇)ofthealveolus,theV ̇A/Q ̇ iszero.Or,at the other extreme, when there is adequate ventilation (V ̇ A) but zeroperfusion(Q ̇),theratioV ̇A/Q ̇ isinfinity.Ataratioofei- ther zero or infinity, there is no exchange of gases through the respiratory membrane of the affected alveoli. Therefore, let us explain the respiratory consequences of these two extremes.
Alveolar Oxygen and Carbon Dioxide Partial Pres- sures When V ̇ A /Q ̇ Equals Zero. When V ̇ A /Q ̇ is equal to zero—that is, without any alveolar ventilation—the air in the alveolus comes to equilibrium with the blood O2 and CO2 because these gases diffuse between the blood and alveolar air. Because the blood that perfuses the capillaries is venous blood returning to the lungs from the systemic circulation, it is the gases in this blood with which the alveolar gases equilibrate. In Chapter 41, we describe how the normal venous blood (V ) hasaPo2 of40mmHgandaPco2 of45mmHg.Therefore, these are also the normal partial pressures of these two gases in alveoli that have blood flow but no ventilation.
Alveolar Oxygen and Carbon Dioxide Partial Pressures When V ̇A/Q ̇ Equals Infinity. The effect on the alveolar gas partial pressures when V ̇ A/Q ̇ equals infinity is entirely dif- ferent from the effect when V ̇ A/Q ̇ equals zero because now there is no capillary blood flow to carry O2 away or to bring CO2 to the alveoli. Therefore, instead of the alveolar gases coming to equilibrium with the venous blood, the alveolar air becomes equal to the humidified inspired air. That is, the air that is inspired loses no O2 to the blood and gains no CO2 from the blood. Furthermore, because normal inspired and humidified air has a Po2 of 149 mm Hg and a Pco2 of 0 mm Hg, these will be the partial pressures of these two gases in the alveoli.
Gas Exchange and Alveolar Partial Pressures When V ̇A/Q ̇ Is Normal. When there is both normal alveolar ventilation and normal alveolar capillary blood flow (normal alveolar perfusion), exchange of O2 and CO2 through the respiratory membrane is nearly optimal, and alveolar Po2 is normally at a level of 104 mm Hg, which lies between that of the inspired air (149 mm Hg) and that of venous blood (40 mm Hg). Likewise, alveolar Pco2 lies between two extremes; it is normally 40 mm Hg, in contrast to 45 mm Hg in venous blood and 0 mm Hg in inspired air. Thus, under normal conditions, the alveolar air Po2 averages 104 mm Hg and the Pco2 aver- ages 40 mm Hg.
Po2-Pco2, V ̇A/Q ̇ Diagram
The concepts presented in the preceding sections are shown in graphic form in Figure 40-11, called the Po2- Pco2, V ̇ A/Q ̇ diagram. The curve in the diagram represents all possible Po2 and Pco2 combinations between the limits of V ̇ A/Q ̇ equals zero and V ̇ A/Q ̇ equals infinity when the gas pressures in the venous blood are normal, and the person is breathing air at sea level pressure. Thus, point V ̇ A/Q ̇ is the plot of Po2 and Pco2 when V ̇ A/Q ̇ equals zero. At this point,the Po2 is 40 mm Hg, and the Pco2 is 45 mm Hg, which are the values in normal venous blood. ̇ ̇
At the other end of the curve, when VA/Q equals infin- ity, point I represents inspired air, showing Po2 to be 149 mm Hg while Pco2 is zero. Also plotted on the curve is the point that represents normal alveolar air when V ̇ A/Q ̇ is normal. At this point, Po2 is 104 mm Hg, and Pco2 is 40 mm Hg.
Concept of Physiological Shunt (When V ̇A/Q ̇ Is Below Normal)
Whenever V ̇ A/Q ̇ is below normal, there is inadequate ven- tilation to provide the O2 needed to fully oxygenate the blood flowing through the alveolar capillaries. Therefore, a certain fraction of the venous blood passing through the pulmonary capillaries does not become oxygenated. This fraction is called shunted blood. Also, some additional blood flows through bronchial vessels rather than through alveolar capillaries, normally about 2% of the cardiac out- put; this, too, is unoxygenated, shunted blood.
The total quantitative amount of shunted blood per min- ute is called the physiological shunt. This physiological shunt is measured in clinical pulmonary function laboratories by analyzing the concentration of O2 in both mixed venous blood and arterial blood, along with simultaneous measure- ment of cardiac output. From these values, the physiological shunt can be calculated by the following equation:
Q ̇PS =CiO2 −CaO2 Q ̇T CiO2 −CvO2
in which Q ̇ PS is the physiological shunt blood flow per minute, Q ̇ T is cardiac output per minute, CiO2 is the concen- tration of oxygen in the arterial blood if there is an “ideal” ventilation-perfusion ratio, CaO2 is the measured concen- tration of oxygen in the arterial blood, and Cv_O2 is the meas- ured concentration of oxygen in the mixed venous blood.
The greater the physiological shunt, the greater the amount of blood that fails to be oxygenated as it passes through the lungs.
Concept of Physiological Dead Space When V ̇A/Q ̇ Greater Than Normal
When ventilation of some of the alveoli is great but alveo- lar blood flow is low, there is far more available oxygen in the alveoli than can be transported away from the alveoli by the flowing blood. Thus, the ventilation of these alve- oli is said to be wasted. The ventilation of the anatomical dead space areas of the respiratory passageways is also wasted. The sum of these two types of wasted ventila- tion is called the physiological dead space. This space is measured in the clinical pulmonary function laboratory by making appropriate blood and expiratory gas meas- urements and by using the following equation, called the Bohr equation:
V ̇ Dphys = PaCO2 − PeCO2 V ̇ T PaCO2
in which V ̇ Dphys is the physiological dead space, V ̇ T is the tidal volume, Paco2 is the partial pressure of CO2 in the arterial blood, and Pe_co2 is the average partial pressure of CO2 in the entire expired air.
When the physiological dead space is great, much of the work of ventilation is wasted effort because so much of the ventilating air never reaches the blood.
Abnormalities of Ventilation-Perfusion Ratio
Abnormal V ̇ A /Q ̇ in Upper and Lower Normal Lung. In a healthy person in the upright position, both pulmonary capillary blood flow and alveolar ventilation are consider- ably less in the upper part of the lung than in the lower part; however, the decrease of blood flow is considerably greater than the decrease in ventilation. Therefore, at the top of the lung, V ̇ A/Q ̇ is as much as 2.5 times as great as the ideal value, which causes a moderate degree of physiologi- cal dead space in this area of the lung.
At the other extreme, at the bottom of the lung, there is slightly too little ventilation in relation to blood flow, with V ̇ A/Q ̇ as low as 0.6 times the ideal value. In this area, a small fraction of the blood fails to become normally oxy- genated, and this represents a physiological shunt.
In both extremes, inequalities of ventilation and perfu- sion slightly decrease the lung’s effectiveness for exchang- ing O2 and CO2. However, during exercise, blood flow to the upper part of the lung increases markedly, so far less physiological dead space occurs, and the effectiveness of gas exchange now approaches optimum.
Abnormal V ̇A/Q ̇ in Chronic Obstructive Lung Disease
Most people who smoke for many years develop various degrees of bronchial obstruction; in many of them, this condition eventually becomes so severe that serious al- veolar air trapping develops, with resultant emphysema. The emphysema, in turn, causes many of the alveolar walls to be destroyed. Thus, two abnormalities occur in smok- ers to cause abnormal V ̇ A/Q ̇ . First, because many of the small bronchioles are obstructed, the alveoli beyond the obstructions are unventilated, causing a V ̇ A /Q ̇ that ap- proaches zero. Second, in the areas of the lung where the alveolar walls have mainly been destroyed but there is still alveolar ventilation, most of the ventilation is wasted because of inadequate blood flow to transport the blood gases.
Thus, in chronic obstructive lung disease, some areas of the lung exhibit serious physiological shunt, and other areas exhibit serious physiological dead space. Both conditions tremendously decrease the effectiveness of the lungs as gas exchange organs, sometimes reducing their effectiveness to as little as one-tenth normal. In fact, this condition is the most prevalent cause of pulmonary disability today.
コメント